Turbine Blade 3D Printing with Superalloys
Table des matières
Introduction: Revolutionizing Turbine Blade Manufacturing with Metal Additive Manufacturing
Turbine blades are the heart of performance in many critical industries, from the soaring heights of aerospace to the demanding environments of power generation. These meticulously engineered components operate under extreme conditions, enduring intense heat, high pressures, and significant mechanical stress. Traditionally, manufacturing turbine blades involved complex, multi-step processes like investment casting and precision forging followed by extensive machining. While effective, these methods often face limitations in design complexity, material waste (particularly with expensive superalloys), and long lead times, impacting both innovation cycles and supply chain responsiveness.
Enter metal additive manufacturing (AM), more commonly known as metal L'impression 3D. This transformative technology is rapidly changing the landscape of high-performance component production. Instead of subtracting material from a solid block or casting it into a mold, AM builds parts layer by layer directly from digital designs using high-quality metal powders. For turbine blades, this opens up unprecedented possibilities. Imagine blades with intricate internal cooling channels optimized for maximum heat dissipation, designs achieving unparalleled aerodynamic efficiency, or the ability to produce replacement parts on demand, drastically reducing downtime.
The synergy between advanced superalloys – materials specifically designed for high-temperature strength and creep resistance – and the geometric freedom offered by impression 3D de métaux is particularly potent for turbine blade applications. Nickel-based superalloys like IN738LC, IN718, and Rene 41 possess the exceptional properties required to withstand the harsh operating environments inside jet engines and industrial gas turbines. Metal AM allows engineers to leverage these materials fully, creating components that were previously impossible or prohibitively expensive to manufacture.
For engineers and procurement managers in aerospace, energy, and industrial sectors, understanding the capabilities of metal AM for turbine blade production is no longer optional; it’s a strategic imperative. This technology offers pathways to:
- Amélioration des performances : Optimize designs for better efficiency, durability, and thermal management.
- Réduction des délais d'exécution : Accelerate prototyping and production, enabling faster development cycles and quicker MRO (Maintenance, Repair, and Overhaul).
- Utilisation améliorée des matériaux : Minimize waste of costly superalloys through near-net-shape manufacturing.
- Supply Chain Agility: Enable on-demand production, reduce inventory costs, and mitigate risks associated with traditional supply chains.
- Consolidation partielle : Combine multiple components into a single printed part, reducing assembly time and potential failure points.
Companies like Metal3DP Technology Co., LTD (Met3dp) are at the forefront of this revolution. Headquartered in Qingdao, China, Met3dp specializes in providing comprehensive additive manufacturing solutions, encompassing industry-leading 3D printing equipment and premium, high-performance metal powders tailored for demanding applications. Our expertise in processing challenging materials like superalloys, combined with advanced powder production techniques like gas atomization, ensures the quality and reliability required for mission-critical parts. This article delves into the specifics of using metal AM, particularly with superalloys like IN738LC, IN718, and Rene 41, for manufacturing state-of-the-art turbine blades, guiding you through applications, advantages, material considerations, and more. Whether you are exploring prototyping, serial production, or optimizing your supply chain for critical components, understanding this technology is key to maintaining a competitive edge.
Core Applications: Where are 3D Printed Turbine Blades Deployed?
The unique capabilities of metal additive manufacturing, especially when combined with high-performance superalloys, make it an increasingly attractive solution for producing turbine blades across a range of demanding industries. The ability to create complex geometries, optimize performance, and respond quickly to MRO needs drives its adoption in critical applications where failure is not an option. Key deployment areas include:
1. Aerospace Gas Turbine Engines: This is arguably the most prominent and demanding application. Turbine blades in jet engines operate at extreme temperatures (often exceeding the melting point of the alloy itself, requiring sophisticated cooling) and rotational speeds.
- High-Pressure Turbine (HPT) Blades: These blades experience the hottest gas flow directly after the combustor. AM allows for the creation of highly intricate internal cooling channels (serpentine passages, micro-channels, film cooling holes) that are extremely difficult or impossible to achieve with traditional casting. This enhanced cooling allows engines to run hotter, increasing efficiency and thrust. Superalloys like IN738LC and specialized single-crystal alloys (often developed proprietarily) are common here.
- Low-Pressure Turbine (LPT) Blades: While operating at lower temperatures than HPT blades, LPT blades are larger and subject to significant centrifugal forces. AM enables weight reduction through optimized internal structures and topology optimization, improving overall engine efficiency and potentially reducing stress on downstream components. Materials like IN718 are frequently used.
- Compressor Blades: Though typically operating at lower temperatures than turbine blades, some advanced compressor stages also benefit from the design freedom and material options offered by AM, especially for lightweighting or complex aerodynamic profiles.
- MRO and Legacy Systems: AM provides a vital solution for producing replacement blades for aging aircraft fleets where original tooling may no longer exist or minimum order quantities for casting are prohibitive. This ensures continued airworthiness and operational readiness, a key concern for defense and commercial aviation procurement teams.
2. Industrial Gas Turbines (IGTs) for Power Generation: Similar to jet engines but typically larger and designed for longevity and efficiency over sheer thrust, IGTs are crucial for electricity generation.
- Pales et aubes de turbines : AM enables the production of large, complex blades with advanced cooling designs to boost turbine efficiency and output. The ability to rapidly produce customized or upgraded blade designs allows power plant operators to improve performance during maintenance cycles. Superalloys like IN738LC and IN718 are workhorses in this sector due to their excellent balance of strength, creep resistance, and corrosion resistance in combustion environments.
- Burner Components and Fuel Nozzles: While not blades, related hot-section components also benefit significantly from AM’s ability to create complex internal passages for optimal fuel-air mixing and cooling, improving combustion efficiency and reducing emissions.
- Réparation et remise à neuf : AM techniques like Direct Energy Deposition (DED) or Laser Metal Deposition (LMD) are increasingly used to repair worn or damaged IGT blades, often adding material back to worn tips or leading edges, significantly extending component life and reducing MRO costs for power generation suppliers.
3. Steam Turbines: Used in power generation (often in conjunction with nuclear or fossil fuel heat sources) and large-scale industrial drives, steam turbines operate under different conditions (high pressure, moisture) than gas turbines.
- Blade Optimization: While superalloys are less common here (stainless steels and titanium alloys are more typical), AM can still offer advantages in optimizing blade profiles for specific steam conditions, improving efficiency, especially in the last stages where blades are very long and complex.
- Prototypage rapide : Testing new aerodynamic designs is faster and more cost-effective with AM compared to traditional methods.
4. High-Performance Turbochargers: Found in automotive and marine applications, turbochargers use a small turbine to drive a compressor, boosting engine power and efficiency.
- Turbine Wheels: The turbine wheel operates at high temperatures and speeds. While often made from materials like Inconel (e.g., IN713C via casting), AM offers potential for creating lighter wheels with optimized blade geometries using materials like IN718 or even titanium aluminides (TiAl) for extreme performance applications, improving transient response.
Meeting B2B Needs: For procurement managers and supply chain specialists, metal AM offers a compelling value proposition in these applications:
- Reduced Tooling Costs: Eliminates the need for expensive casting molds or forging dies, especially beneficial for low-to-medium volume production runs or legacy parts.
- On-Demand Availability: Facilitates digital inventory and localized production, reducing reliance on distant suppliers and long lead times for critical spares.
- Customization and Upgrades: Enables tailored designs for specific performance requirements or integration of design improvements without major retooling investments.
- Supplier Consolidation: Partnering with a capable AM provider like Met3dp, offering both advanced printing services and high-quality powder supply, can streamline the procurement process for complex components.
The deployment of 3D printed turbine blades is rapidly expanding as the technology matures, demonstrating tangible benefits in performance, cost, and supply chain resilience across these vital industrial sectors.
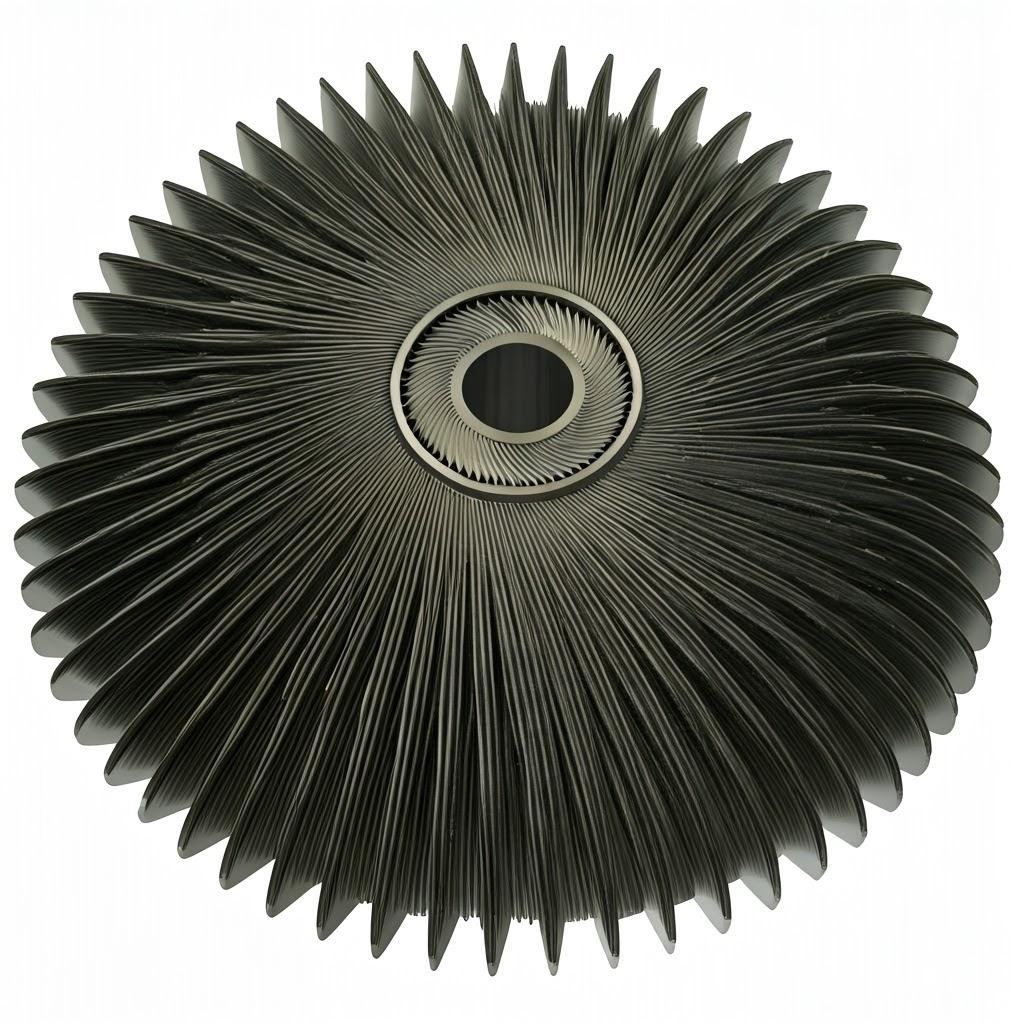
The AM Advantage: Why Choose Metal 3D Printing for Turbine Blade Production?
While traditional manufacturing methods like investment casting and multi-axis CNC machining have served the industry well for decades, metal additive manufacturing presents a paradigm shift, offering distinct advantages crucial for the demanding requirements of turbine blade production. These benefits address key challenges faced by engineers and procurement professionals, impacting design, lead times, cost, and overall supply chain efficiency.
1. Unprecedented Design Freedom: This is perhaps the most significant advantage. Traditional methods are constrained by tooling requirements (e.g., draft angles for casting, tool access for machining). AM builds parts layer-by-layer, freeing designers from many of these constraints.
- Complex Internal Geometries: Enables the creation of highly sophisticated internal cooling channels within blades (e.g., serpentine passages, lattice structures, conformal cooling) that are impossible or impractical to achieve otherwise. Better cooling allows for higher turbine inlet temperatures, directly translating to increased engine/turbine efficiency and power output.
- Optimisation de la topologie : Software can optimize the blade’s structure, removing material from low-stress areas while reinforcing critical regions. This leads to lighter blades without compromising strength, reducing rotational mass and improving engine response and fuel efficiency.
- Consolidation partielle : Multiple components (e.g., a blade and its platform or shroud elements) can potentially be printed as a single unit, reducing assembly steps, weight, part count, and potential points of failure.
- Biomimicry & Novel Designs: AM allows exploration of entirely new aerodynamic shapes and internal structures inspired by nature or advanced computational fluid dynamics (CFD) simulations.
2. Accelerated Development and Lead Time Reduction: Traditional manufacturing involves long lead times for tooling creation (months for complex casting molds) and setup. AM significantly shortens the path from design to physical part.
- Prototypage rapide : Engineers can design, print, and test functional prototypes in days or weeks, rather than months. This allows for more design iterations and faster optimization cycles.
- Tooling Elimination: AM is a tool-less process. This drastically cuts the initial lead time and cost associated with producing molds or dies, especially beneficial for low-volume production or customized parts.
- On-Demand Production & MRO: Spare or replacement blades can be printed as needed, minimizing inventory holding costs and reducing Aircraft on Ground (AOG) time or power plant downtime. This agility is critical for efficient MRO operations and resilient supply chains.
3. Enhanced Material Efficiency: Superalloys used for turbine blades (Inconels, Rene alloys) are notoriously expensive. Traditional subtractive manufacturing can waste a significant amount of this valuable material (high buy-to-fly ratio).
- Near-Net Shape Manufacturing: AM processes like Selective Laser Melting (SLM) or Electron Beam Melting (EBM) build parts layer by layer, using only the material necessary for the part and its support structures. This dramatically reduces material waste compared to machining from a solid billet.
- Optimized Material Use: Topology optimization inherently designs parts using material only where structurally required, further enhancing material efficiency.
4. Potential for Cost-Effectiveness (Context Dependent): While the cost per part in AM can sometimes be higher than mass-produced cast parts, the overall value proposition is often compelling:
- Reduced Tooling Investment: Eliminates high upfront tooling costs, making it economical for prototypes, low-volume series, and customized parts.
- Lower Assembly Costs: Achieved through part consolidation.
- Reduced Material Waste Costs: Significant savings, especially with expensive superalloys.
- Lower Inventory Costs: Enabled by on-demand production.
- Value of Performance Gains: Increased efficiency or longevity achieved through superior AM designs can outweigh higher initial part costs over the component’s lifecycle (Total Cost of Ownership).
5. Supply Chain Simplification and Resilience: AM enables a shift towards digital manufacturing.
- Digital Inventory: Designs are stored digitally and can be printed anywhere with the right equipment and certified processes/materials.
- Production localisée : Reduces reliance on complex global supply chains, mitigating geopolitical risks and transportation costs/delays.
- Reduced Supplier Dependency: Less reliance on specialized casting foundries, potentially broadening the supplier base.
Comparison Summary: AM vs. Traditional Methods for Turbine Blades
Fonctionnalité | Metal Additive Manufacturing (SLM/EBM) | Moulage à la cire perdue | CNC Machining (from billet/forging) |
---|---|---|---|
Complexité de la conception | Very High (complex internal features possible) | Moderate (limited by mold design) | Moderate (limited by tool access) |
Internal Cooling | Excellent (highly complex channels feasible) | Good (established techniques exist) | Poor (very difficult/impossible) |
Lead Time (Initial) | Short (days/weeks – no hard tooling) | Long (months – mold production) | Moderate (weeks – setup/programming) |
Lead Time (Repeat) | Modéré | Short-Moderate (using existing mold) | Modéré |
Déchets matériels | Low (near-net shape) | Moderate (gates, runners) | High (significant chip removal) |
Coût de l'outillage | None/Minimal (supports) | High (mold design & production) | Low-Moderate (fixtures, tools) |
Cost (Low Volume) | Potentially Lower (no tooling amortization) | High (tooling cost dominates) | High (material waste, machine time) |
Cost (High Volume) | Potentially Higher (slower build rate) | Lower (amortized tooling, faster cycle) | Modéré-élevé |
Consolidation partielle | High Potential | Low Potential | Low Potential |
Options de matériaux | Growing (requires powder development & process params) | Wide (well-established) | Wide (requires machinable stock) |
Exporter vers les feuilles
Choosing metal AM, supported by expert providers like Met3dp who understand both the advanced méthodes d'impression and material science, empowers businesses to overcome traditional limitations and unlock new levels of performance and efficiency in turbine blade production. Procurement teams benefit from reduced lead times, enhanced supply chain flexibility, and the potential for long-term cost savings through superior component design and lifecycle.
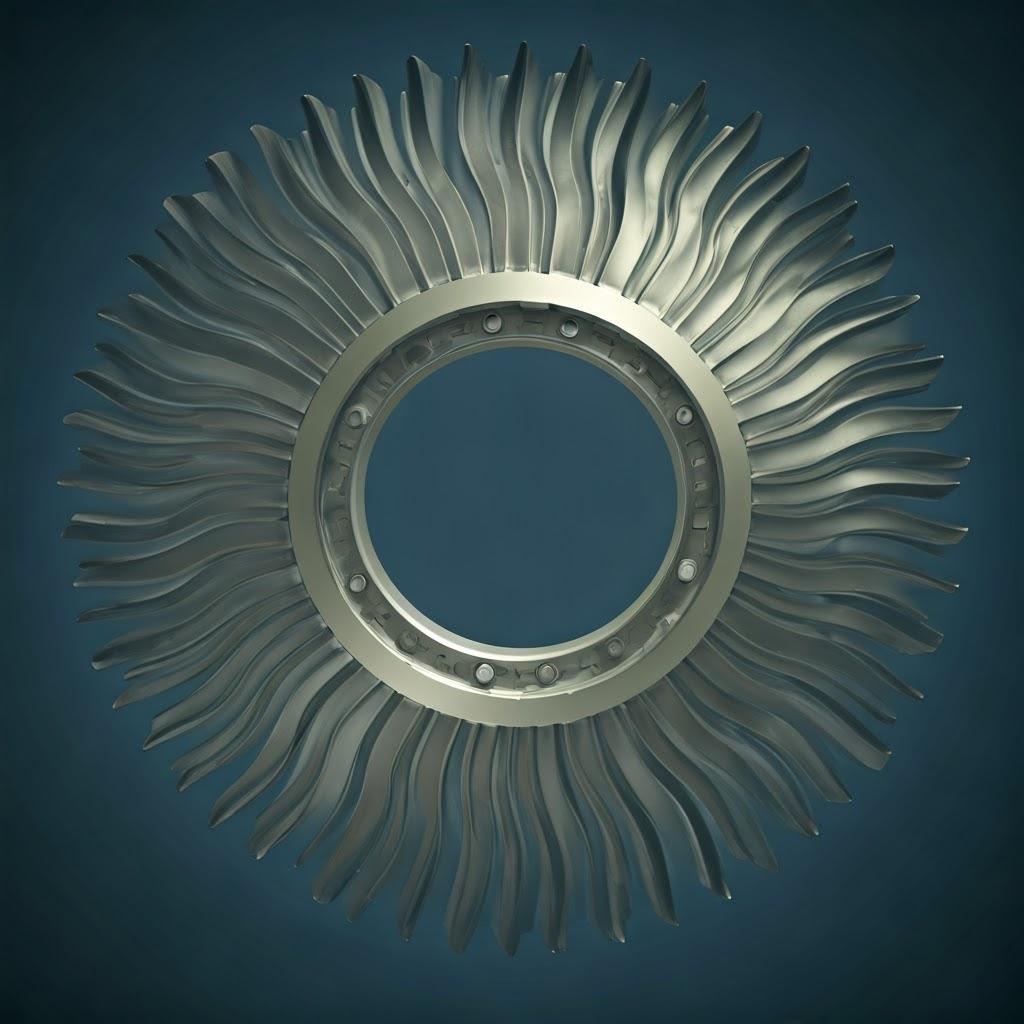
Material Focus: IN738LC, IN718, Rene 41 Superalloys for High-Performance Blades
The extreme operating conditions within gas turbines necessitate the use of materials with exceptional properties. Superalloys, typically nickel-based, are the materials of choice for turbine blades due to their ability to maintain high strength, creep resistance, fatigue life, and surface stability (resistance to oxidation and corrosion) at elevated temperatures approaching their melting points. Selecting the right superalloy and ensuring its quality in powdered form is paramount for successful additive manufacturing of reliable turbine components. Among the most relevant and frequently used superalloys printable via AM for these applications are IN738LC, IN718, and Rene 41.
Why Superalloys? Before diving into specifics, it’s crucial to understand why these materials are essential:
- Résistance à haute température : They retain significant tensile and yield strength even when exposed to temperatures exceeding 800°C (1472°F), and often much higher for short durations or with cooling.
- Résistance au fluage : They resist slow deformation under constant stress at high temperatures, critical for blades under centrifugal load in a hot gas path.
- Résistance à la fatigue : They withstand cyclic loading (thermal and mechanical) experienced during turbine start-up, operation, and shutdown.
- Corrosion & Oxidation Resistance: They form protective oxide layers (typically chromia or alumina) to resist degradation from hot combustion gases and environmental factors.
Recommended Superalloys for AM Turbine Blades:
1. Inconel 738 Low Carbon (IN738LC):
- Vue d'ensemble : A precipitation-hardenable nickel-based superalloy known for its excellent high-temperature strength, creep resistance, and hot corrosion resistance, particularly in sulfur-containing environments typical of industrial gas turbines. The “LC” variant has lower carbon content for improved weldability and, consequently, better printability compared to standard IN738.
- Propriétés principales : Excellent creep strength up to ~980°C (1800°F), good castability (historically), and good resistance to hot corrosion. Requires specific heat treatments after printing to achieve optimal microstructure and properties.
- AM Considerations: Can be challenging to print due to its susceptibility to solidification cracking if parameters are not carefully controlled. Requires precise thermal management during the build process and often benefits from Hot Isostatic Pressing (HIP) post-processing to close internal porosity.
- Applications typiques : Primarily used for first-stage turbine blades and vanes in industrial gas turbines (IGTs) due to its superior hot corrosion resistance and high creep strength.
2. Inconel 718 (IN718):
- Vue d'ensemble : One of the most widely used nickel-based superalloys due to its good balance of properties, excellent fabricability (including printability), and relatively lower cost compared to other high-end superalloys. It is precipitation-strengthened by niobium and molybdenum additions.
- Propriétés principales : High strength up to ~700°C (1300°F), good creep resistance up to that temperature, excellent weldability/printability, good fatigue strength, and good corrosion resistance. Readily available and well-characterized for AM processes.
- AM Considerations: Generally considered one of the easier superalloys to print using Laser Powder Bed Fusion (L-PBF/SLM) and Electron Beam Melting (EBM). Standard heat treatments (solution annealing and aging) are well-established to develop desired properties.
- Applications typiques : Widely used in aerospace for compressor disks and blades, engine casings, low-pressure turbine (LPT) blades, and various structural components. Also used in IGTs for components operating at slightly lower temperatures, as well as in turbochargers, nuclear applications, and chemical processing.
3. Rene 41 (Haynes® R-41):
- Vue d'ensemble : An age-hardenable nickel-based superalloy known for its very high strength at elevated temperatures. It contains significant amounts of chromium, cobalt, and molybdenum, contributing to its strength and corrosion resistance.
- Propriétés principales : Exceptionally high strength up to ~870°C (1600°F). Good oxidation resistance.
- AM Considerations: Can be very challenging to process via AM due to its high susceptibility to strain-age cracking during post-weld or post-print heat treatments if not handled carefully. Requires meticulously developed print parameters and often specialized heat treatment cycles. Its printability is generally considered lower than IN718.
- Applications typiques : Used in demanding aerospace applications requiring high strength-to-weight ratios at high temperatures, such as afterburner components, turbine casings, bolts, and some turbine blade/vane applications where its specific strength profile is advantageous, despite processing challenges.
Comparative Overview:
Fonctionnalité | IN738LC | IN718 | Rene 41 (Haynes® R-41) |
---|---|---|---|
Primary Strengths | Hot Corrosion Resistance, High Creep Str. | Printability, Balanced Properties, Cost | Very High Temp. Strength |
Max Use Temp (approx) | ~980°C (1800°F) – Creep limited | ~700°C (1300°F) – Strength limited | ~870°C (1600°F) – Strength limited |
Imprimabilité | Moderate (crack sensitive) | Excellent | Difficult (strain-age crack sensitive) |
Post-traitement | Heat Treatment, Often HIP | Standard Heat Treatment, HIP optional | Specialized Heat Treatment, HIP often req. |
Typical AM Use | IGT Blades/Vanes | Aerospace Components, LPT Blades, IGT Parts | Aerospace Hot Sections, High-Stress Parts |
Exporter vers les feuilles
The Critical Role of Powder Quality: Successful additive manufacturing of turbine blades hinges not just on selecting the right alloy, but on the quality of the metal powder feedstock. Key powder characteristics impacting printability and final part properties include:
- Sphéricité : Highly spherical particles ensure good powder flowability and uniform powder bed density, leading to consistent melting and reduced porosity.
- Distribution de la taille des particules (PSD) : A controlled PSD is crucial for achieving high packing density and predictable melting behavior. Fines can affect flowability and potentially pose safety hazards, while overly large particles may not melt completely.
- Fluidité : Ensures uniform spreading of powder layers by the recoater mechanism in the printer, preventing voids and defects.
- La pureté : Low levels of impurities (like oxygen, nitrogen) and absence of contaminants are essential to prevent defects and ensure the desired mechanical properties and corrosion resistance of the final part. Satellite particles (smaller particles attached to larger ones) should be minimized.
- Composition chimique : Must strictly adhere to the alloy specification to guarantee performance.
Met3dp’s Commitment to Powder Excellence: Recognizing the critical importance of powder quality, Met3dp employs industry-leading manufacturing technologies to produce superior metal powders for additive manufacturing.
- Advanced Atomization: Utilizing state-of-the-art Vacuum Induction Melting Gas Atomization (VIGA) and Plasma Rotating Electrode Process (PREP) systems, Met3dp produces powders with exceptional sphericity, low satellite content, and high purity. Our unique nozzle and gas flow designs in gas atomization ensure optimized particle morphology.
- Un contrôle de qualité rigoureux : Every batch of powder undergoes stringent testing for chemical composition, PSD, flowability, density, and morphology to ensure it meets the demanding specifications required for critical applications like turbine blades.
- Wide Portfolio: Beyond IN738LC, IN718, and Rene 41 (availability may vary, please inquire), Met3dp offers a broad range of high-performance metal powders, including titanium alloys, cobalt-chrome, stainless steels, and innovative alloys, supporting diverse industrial needs. You can explore our range of metal powder products here.
Choosing the right superalloy powder and ensuring its quality is a foundational step for procurement managers and engineers aiming to leverage AM for high-performance turbine blades. Partnering with a knowledgeable supplier like Met3dp, with proven expertise in both powder production and AM processes, provides the confidence needed for manufacturing mission-critical components.
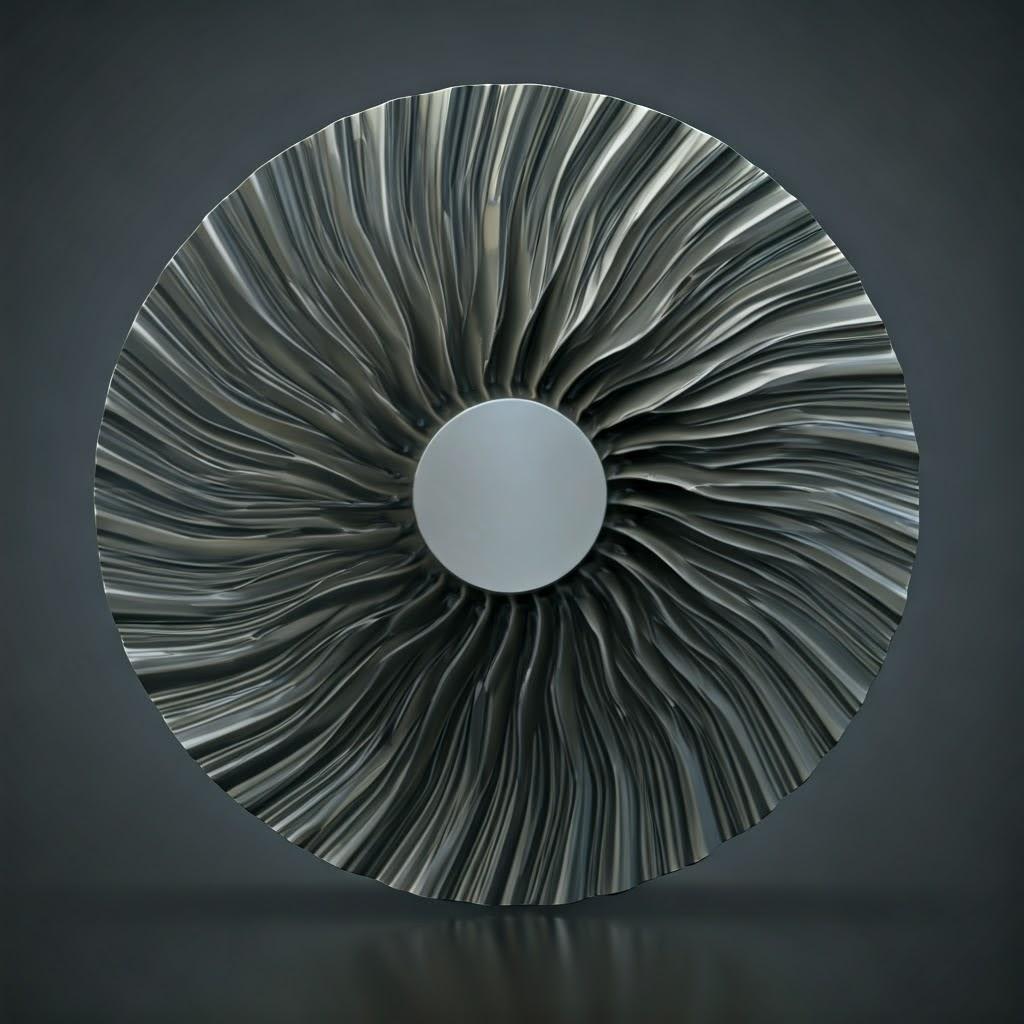
Design for Additive Manufacturing (DfAM): Optimizing Turbine Blade Geometry</h2>
Simply replicating a turbine blade design originally intended for casting or machining using additive manufacturing often fails to capture the true potential of the technology. To fully harness the benefits of metal 3D printing – enhanced performance, reduced weight, and improved manufacturability – engineers must embrace Design for Additive Manufacturing (DfAM). DfAM is not just a set of rules but a mindset shift, focusing on designing parts specifically for the layer-by-layer AM process, considering its unique capabilities and constraints, especially when dealing with high-performance superalloys.
Why DfAM is Crucial for Turbine Blades: Turbine blades are highly complex, performance-critical components. Applying DfAM principles allows engineers to:
- Maximize Performance: Leverage geometric freedom to create optimized cooling channels and aerodynamic surfaces beyond traditional limits.
- Reduce Weight: Utilize topology optimization and internal lattice structures to minimize mass while maintaining structural integrity, crucial for rotating components.
- Improve Print Success: Design features that minimize residual stress, reduce the need for difficult-to-remove supports, and account for material shrinkage and anisotropy.
- Streamline Post-Processing: Design with finishing operations in mind, ensuring access for machining critical surfaces or removing internal supports.
- Accelerate Innovation: Rapidly iterate designs optimized for AM, testing new concepts quickly and efficiently.
Key DfAM Principles for AM Turbine Blades:
- Advanced Internal Cooling Channel Design:
- Conformal Cooling: Design cooling channels that follow the complex 3D contour of the blade surface, providing more uniform and efficient heat removal compared to straight, drilled holes.
- Géométries complexes : Incorporate features like trip strips, pedestals, and complex branching networks within channels to enhance heat transfer (turbulence) with minimal pressure drop.
- Structures en treillis : Use internal lattice or gyroid structures within larger cavities for lightweighting while providing structural support and potentially aiding heat dissipation.
- Smooth Transitions: Avoid sharp corners in internal channels, which can cause stress concentrations and impede powder removal after printing. Gradual bends are preferred.
- Taille minimale des fonctionnalités : Be mindful of the AM process limitations regarding the smallest channel diameter or wall thickness that can be reliably produced. This varies by machine and material.
- Optimisation de la topologie et allègement :
- Utilize specialized software to define load cases, constraints, and design spaces (e.g., blade platform, shroud attachments). The software then iteratively removes material from non-critical areas, resulting in an organic, load-path-optimized structure.
- This is particularly effective for reducing the weight of the blade’s root section or platform, lessening the centrifugal load on the turbine disk.
- Combine topology optimization with lattice structures for further weight reduction in less critical areas.
- Support Structure Strategy and Self-Supporting Design:
- AM processes typically require support structures for overhanging features (usually below 45 degrees from the horizontal) to anchor them to the build plate or lower layers and dissipate heat.
- Minimize Supports: Design features to be self-supporting (steeper angles, chamfers instead of sharp overhangs) wherever possible, especially on critical aerodynamic surfaces or hard-to-reach internal areas.
- Optimize Support Placement: Place supports strategically to minimize contact points (“witness marks”) on functional surfaces. Use easily removable support types (e.g., conical, perforated).
- Conception pour l'accès : Ensure there is physical access for tools or processes needed to remove supports, particularly complex internal ones.
- Consolidation partielle :
- Explore opportunities to combine the blade airfoil with its platform, shroud elements, or damping features into a single printed component.
- Benefits include reduced part count, elimination of assembly processes (like welding or brazing), potentially lower weight, and removal of interfaces that could be failure points.
- Requires careful consideration of printability, residual stress management in the larger, more complex part, and post-processing accessibility.
- Orientation and Anisotropy Considerations:
- The orientation in which the blade is printed on the build plate significantly impacts:
- Finition de la surface : Different finishes on up-facing, down-facing, and vertical surfaces.
- Support Requirements: Affects the amount and location of supports.
- Propriétés mécaniques : Due to the columnar grain growth typical in many AM processes, properties (like fatigue strength or creep) can differ slightly along the build direction (Z-axis) versus perpendicular to it (X-Y plane).
- DfAM involves selecting the optimal build orientation early in the design process to balance surface finish, support needs, print time, and anisotropic property effects for the specific application requirements.
- The orientation in which the blade is printed on the build plate significantly impacts:
- Thermal Management Features:
- Incorporate design elements that help mitigate the buildup of residual thermal stress during printing.
- Use gradual transitions between thick and thin sections. Avoid large, solid blocks of material where heat can concentrate.
- Consider sacrificial features or optimized support strategies specifically designed to draw heat away effectively.
Engaging with an experienced additive manufacturing partner is invaluable for implementing DfAM effectively. Companies like Met3dp bring not only advanced printing technology but also crucial application development expertise. Understanding the nuances of how design choices interact with specific superalloys (like IN738LC, IN718, Rene 41) and the selected méthodes d'impression is key to success. By integrating DfAM principles from the outset, engineering and procurement teams can ensure they are truly leveraging the transformative power of AM for producing next-generation turbine blades.
Achieving Precision: Tolerance, Surface Finish, and Accuracy in AM Turbine Blades
While metal additive manufacturing offers unparalleled design freedom, it’s crucial for engineers and procurement managers to have realistic expectations regarding the dimensional accuracy, tolerances, and surface finish achievable directly from the printing process, especially for precision components like turbine blades made from superalloys. Understanding these aspects is vital for defining requirements, planning post-processing steps, and ensuring the final part meets stringent performance and assembly criteria.
As-Built Dimensional Accuracy: The accuracy of a part right off the AM machine depends on several factors, including machine calibration, laser/electron beam spot size, layer thickness, material properties (shrinkage, thermal conductivity), scan strategy, and the effectiveness of support structures in managing thermal stresses.
- Typical Ranges: For processes like Laser Powder Bed Fusion (L-PBF/SLM), typical dimensional accuracy might range from ±0.05mm to ±0.2mm (±0.002″ to ±0.008″) for smaller features, potentially increasing slightly over larger part dimensions. Electron Beam Melting (EBM) often has slightly looser tolerances due to its higher processing temperatures and powder sintering effects but excels in reducing residual stress.
- Influencing Factors: Thermal warping during the build is a primary challenge. Careful orientation, robust support structures, and optimized process parameters are essential to minimize deviation from the intended geometry. Post-print stress relief and HIP can also cause minor, predictable dimensional changes.
Surface Finish (Surface Roughness – Ra): The as-built surface finish of AM parts is inherently rougher than that achieved by traditional machining or polishing. This is due to the layer-by-layer nature of the process and the presence of partially melted powder particles adhering to the surface.
- Typical Ra Values:
- L-PBF (SLM): As-built Ra typically ranges from 6µm to 20µm (240µin to 800µin), heavily dependent on the surface orientation relative to the build direction.
- EBM : Generally produces rougher surfaces than L-PBF, often in the Ra 20µm to 40µm (800µin to 1600µin) range, due to larger powder particles and higher temperatures causing some powder sintering.
- Orientation Dependence:
- Up-skin Surfaces: Surfaces facing upwards during the build tend to be smoother.
- Down-skin Surfaces: Surfaces facing downwards, supported by structures, are typically the roughest due to support contact points and heat effects.
- Vertical Walls: Show distinct layer lines, contributing to roughness. Angled surfaces exhibit stair-stepping effects.
- Impact: Surface roughness significantly impacts aerodynamic performance (drag) and can be initiation sites for fatigue cracks. Therefore, critical airfoil surfaces almost always require post-process finishing. Internal cooling channels also need controlled roughness for optimal heat transfer and flow.
Tolerancing (GD&T – Geometric Dimensioning and Tolerancing): For turbine blades, certain features demand very tight tolerances that are often beyond the capability of as-built AM processes.
- Critical Features: These typically include:
- Root Form (e.g., Fir Tree): Must mate precisely with the turbine disk slot. Requires tolerances in the micron range.
- Shroud Interfaces: If applicable, for sealing and damping.
- Tip Clearance: The gap between the blade tip and the casing is critical for efficiency and preventing rubs.
- Leading/Trailing Edges: Profile accuracy affects aerodynamics and stress concentrations.
- AM vs. Final Tolerances: It’s essential to distinguish between achievable as-built tolerances and the final required tolerances. Designers must clearly indicate which features require tighter tolerances that will be achieved through post-machining.
- Specifying for AM: When specifying drawings for AM parts, use GD&T callouts realistically. Indicate as-built tolerances where acceptable and clearly define surfaces/features requiring finishing operations with their final tolerances.
Inspection and Quality Control: Verifying the dimensional accuracy and integrity of AM turbine blades is crucial.
- Machines à mesurer tridimensionnelles (MMT) : Used for precise measurement of critical dimensions and geometric features, often after post-machining.
- 3D Scanning (Laser/Structured Light): Provides a full-field comparison of the printed part against the original CAD model, useful for checking overall form, detecting warpage, and verifying complex airfoil shapes. Can be used on as-built and finished parts.
- CT Scanning: While primarily for internal defect detection (see Post-Processing), it can also provide dimensional data, especially for internal channels.
Implications for Procurement and Engineering:
- Clear Requirements: Define critical dimensions, tolerances, and surface finish requirements unambiguously in RFQs and technical specifications. Differentiate between as-built and final finished states.
- Post-Processing Planning: Recognize that achieving tight tolerances and smooth finishes on critical surfaces will necessitate secondary operations like CNC machining and surface finishing. Factor this into lead time and cost estimations.
- Supplier Capability: Ensure the chosen AM service provider has not only precise printing capabilities but also robust quality control systems and, ideally, integrated post-processing capabilities or strong partnerships for finishing operations. Understanding a supplier’s validated process capability for specific materials and features is key.
Achieving the necessary precision for AM turbine blades is a multi-stage process. While AM provides the initial complex geometry, targeted post-processing is essential for meeting the stringent tolerance and surface finish demands of these critical components.
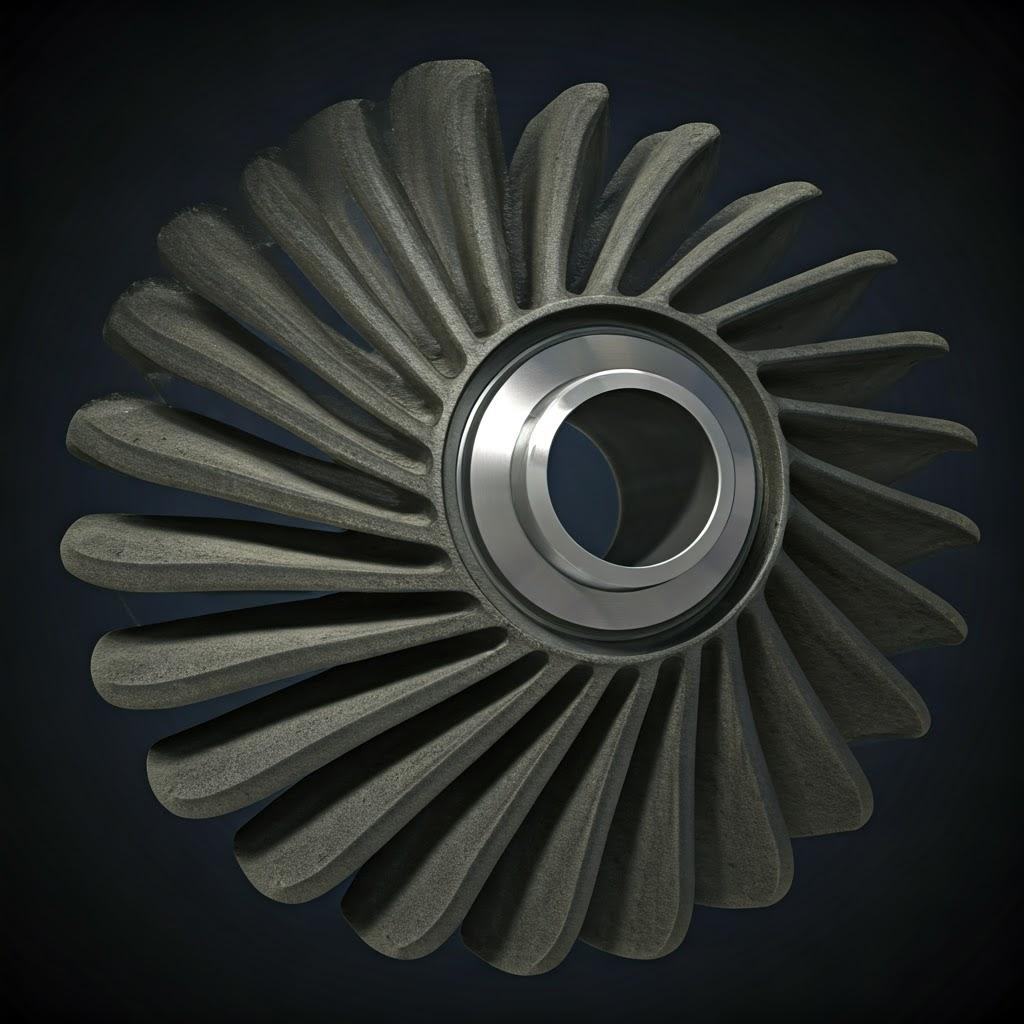
Beyond the Print: Essential Post-Processing for Superalloy Turbine Blades
Producing a geometrically accurate turbine blade using additive manufacturing is a significant achievement, but the journey from the printer to a flight-worthy or operational component is far from over, especially when dealing with high-performance superalloys. A series of critical post-processing steps are required to relieve internal stresses, refine the microstructure, achieve the necessary mechanical properties, meet dimensional tolerances, ensure surface integrity, and verify overall quality. Neglecting or improperly executing these steps can compromise the blade’s performance and potentially lead to premature failure.
Mandatory & Common Post-Processing Steps:
- Traitement thermique anti-stress :
- Objet : To reduce the high residual stresses built up during the rapid heating and cooling cycles inherent in the AM process. These stresses can cause distortion upon removal from the build plate or even cracking.
- Procédure : Typically performed while the part is still attached to the build plate, often in an inert atmosphere furnace. The specific temperature and duration depend on the alloy (e.g., IN718 vs. IN738LC) and part geometry but are generally below the aging temperature.
- Retrait de la pièce de la plaque de construction :
- Méthodes : Commonly done using wire Electrical Discharge Machining (EDM) or a band saw. Care must be taken to avoid damaging the part.
- Retrait de la structure de soutien :
- Objet : To remove the temporary structures required during the build.
- Méthodes : Can range from simple manual breaking (for well-designed supports) to machining, grinding, or EDM for more tenacious or hard-to-reach supports. Internal channel supports often pose the biggest challenge. Advanced techniques like chemical etching or abrasive flow machining may be needed for complex internal passages. DfAM plays a crucial role here.
- Solution Annealing & Aging Heat Treatments:
- Objet : Absolutely critical for precipitation-hardenable superalloys like IN718, IN738LC, and Rene 41. These treatments dissolve inconsistencies from the rapid solidification during AM and then precisely precipitate strengthening phases (like gamma prime and gamma double prime) within the grain structure to achieve the desired high-temperature strength, creep resistance, and fatigue life.
- Procédure : Involves heating to a high solutioning temperature followed by controlled cooling, and then one or more subsequent aging treatments at specific intermediate temperatures for set durations. Cycles are specific to each alloy and must be tightly controlled (temperature uniformity, ramp rates, atmosphere). Improper heat treatment can severely degrade mechanical properties.
- Pressage isostatique à chaud (HIP) :
- Objet : To eliminate internal microporosity (gas pores or lack-of-fusion voids) inherent in the AM process and improve material homogeneity.
- Procédure : The part is subjected to high temperature (below melting point but often near solutioning temperature) and high-pressure inert gas (typically Argon) simultaneously in a specialized HIP vessel. The pressure collapses internal voids, diffusion bonding the material across the void interfaces.
- Avantages : Significantly improves fatigue life, ductility, and toughness. Increases material density closer to theoretical limits. Often mandatory for critical rotating parts in aerospace and IGT applications (considered a densification process). Usually performed after stress relief but before final aging treatments.
- Usinage :
- Objet : To achieve tight dimensional tolerances and specific surface finishes on critical features that cannot be met by the as-built AM process.
- Areas: Typically includes the blade root (fir tree), shroud contact faces, tip surfaces, and sometimes leading/trailing edges or specific airfoil sections.
- Considérations : Requires careful fixture design to hold the complex AM geometry. Machining superalloys is challenging due to their high strength and work-hardening characteristics.
- Finition de la surface :
- Objet : To improve aerodynamic performance by reducing drag and enhance fatigue life by removing surface imperfections that can act as crack initiation sites.
- Méthodes : Varies depending on the required Ra value and accessibility:
- Mass Finishing: Vibratory tumbling, centrifugal finishing (using abrasive media).
- Usinage par flux abrasif (AFM) : Forces abrasive putty through internal channels and across external surfaces – good for complex geometries.
- Electrochemical Polishing (ECM): Removes material electrochemically, producing a very smooth finish.
- Manual Grinding/Polishing: For specific, accessible areas requiring high precision.
- Coating Application:
- Objet : To protect the blade from the extreme temperatures and corrosive environment within the turbine.
- Les types:
- Thermal Barrier Coatings (TBCs): Ceramic coatings (often Yttria-Stabilized Zirconia – YSZ) applied over a metallic bond coat. They insulate the base superalloy, allowing higher gas temperatures.
- Environmental Barrier Coatings (EBCs): Protect against oxidation and corrosion.
- Procédure : Usually applied via plasma spray or electron beam physical vapor deposition (EB-PVD) after final machining and surface preparation.
- Essais non destructifs (END) :
- Objet : To ensure the final part is free from critical internal and external defects après all manufacturing and processing steps.
- Méthodes :
- X-ray Computed Tomography (CT): Provides a 3D view of the internal structure, detecting voids, inclusions, and cracks. Essential for validating internal integrity.
- Contrôle par ressuage fluorescent (FPI) : Détecte les fissures superficielles.
- Contrôle par ultrasons (UT) : Peut détecter des défauts sous la surface.
Integrated Approach: Effective post-processing requires a holistic view, integrating considerations right from the DfAM stage. Procurement managers should ensure potential AM suppliers have established, certified procedures for these critical steps, either in-house or through qualified partners. The cost and lead time associated with post-processing are significant and must be factored into any project plan for producing high-quality, reliable AM superalloy turbine blades. Partnering with a full-service provider like Met3dp, who understands the entire workflow from powder to finished part, can significantly streamline this complex process. Learn more about our comprehensive approach on our À propos de nous page.
Overcoming Hurdles: Common Challenges and Solutions in Superalloy Blade Printing
Additive manufacturing of turbine blades using superalloys like IN738LC, IN718, and Rene 41 is a powerful technology, but it’s not without its challenges. The combination of complex geometries, demanding materials prone to high-temperature phenomena, and the layer-wise fusion process can lead to specific defects or issues if not properly managed. Recognizing these potential hurdles and implementing effective solutions is crucial for consistently producing high-quality, reliable components.
1. Residual Stress, Distortion, and Warping:
- Défi: The rapid, localized heating and cooling cycles during L-PBF or EBM create steep thermal gradients within the part and between the part and the build plate. This generates internal residual stresses that can cause the part to distort, warp (especially thin sections), or even detach from the build plate during the print.
- Solutions :
- Stratégies d'analyse optimisées : Using techniques like island scanning, sector scanning, or varying scan vectors between layers helps distribute heat more evenly and reduce localized stress buildup.
- Structures de soutien robustes : Well-designed supports anchor the part securely and act as heat sinks, drawing thermal energy away more effectively.
- Build Plate Heating: Pre-heating the build plate (common in EBM, increasingly used in L-PBF for crack-sensitive alloys) reduces the thermal gradient between the solidified material and the plate/powder bed.
- Simulation de processus : Utilizing software to predict thermal gradients and stress accumulation allows for optimization of orientation, supports, and scan strategies before printing.
- In-process Stress Relief: Some advanced systems incorporate methods to manage stress during the build.
- Post-Build Stress Relief: Performing a stress relief heat treatment before removing the part from the plate is standard practice.
2. Cracking:
- Défi: Certain superalloys, particularly precipitation-hardenable ones with wide freezing ranges or those susceptible to grain boundary liquation (like IN738LC, Rene 41, and sometimes IN718 under non-optimal conditions), are prone to various forms of cracking either during solidification or subsequent heat treatments.
- Solidification Cracking: Occurs in the melt pool during solidification due to thermal stresses pulling apart dendrites before fusion is complete.
- Liquation Cracking: Occurs in the heat-affected zone (HAZ) adjacent to the melt pool, where low-melting-point phases at grain boundaries can re-melt and crack under stress.
- Strain-Age Cracking: Occurs during post-print heat treatment (aging) in some alloys (notably Rene 41) when residual stresses cause precipitation hardening at grain boundaries, leading to embrittlement and cracking.
- Solutions :
- Careful Parameter Development: Precise control over laser/beam power, scan speed, layer thickness, and hatch spacing is critical to manage the melt pool size and cooling rate. Often requires extensive Design of Experiments (DoE).
- Alloy Selection/Modification: Using variants designed for better weldability/printability (e.g., IN738LC vs. standard IN738).
- Optimized Heat Treatments: Specific stress relief and annealing cycles designed to minimize cracking susceptibility during subsequent aging treatments. HIP can sometimes help heal micro-cracks.
- Substrate Material & Heating: Matching substrate thermal properties and using sufficient pre-heating.
3. Porosity:
- Défi: Small voids within the printed material can act as stress concentrators, significantly reducing fatigue life and mechanical properties. Porosity can arise from:
- Porosité du gaz : Trapped gas (e.g., Argon shielding gas, dissolved gases in powder) forming bubbles in the melt pool that get frozen in place.
- Porosité de manque de fusion : Insufficient energy input or poor powder layer density leading to incomplete melting between layers or adjacent scan tracks.
- Solutions :
- Poudre de haute qualité : Using powder with high sphericity, good flowability, controlled PSD, and low internal gas content (like those produced using Met3dp’s advanced atomization) is fundamental. Proper powder handling and storage are also key.
- Paramètres de processus optimisés : Ensuring sufficient energy density (Power/Speed*Hatch Spacing) to fully melt the material without excessive vaporization or keyholing (which can trap gas).
- Controlled Atmosphere: Maintaining a pure inert gas atmosphere in the build chamber to minimize contamination and gas pickup.
- Pressage isostatique à chaud (HIP) : Highly effective at closing both gas and lack-of-fusion pores, leading to near-fully dense parts. Often considered mandatory for fatigue-critical turbine blades.
4. Support Removal Difficulty:
- Défi: Removing support structures, especially dense or complex ones located within internal cooling channels or on delicate features, can be time-consuming, costly, and risk damaging the part.
- Solutions :
- Focus DfAM : Designing for self-support, optimizing orientation, using easily removable support types (lower density, conical points), and ensuring access for removal tools.
- Techniques avancées de suppression : Utilizing Wire EDM, electrochemical machining, abrasive flow machining, or chemical etching for hard-to-reach areas.
- Optimisation des paramètres du processus : Fine-tuning support parameters for easier detachment without compromising build stability.
5. Anisotropy and Microstructure Control:
- Défi: The directional solidification inherent in AM often leads to columnar grain growth aligned with the build direction (Z-axis). This results in anisotropic mechanical properties (different strength/ductility in Z vs. X/Y directions). Controlling grain structure (e.g., achieving equiaxed grains for better isotropic properties) can be difficult.
- Solutions :
- Understanding Material Behavior: Characterizing anisotropic properties and designing accordingly (e.g., orienting the blade so critical stresses align with the strongest grain direction).
- Process Modifications: Techniques like beam oscillation, pulsed beams, or varying scan strategies can sometimes influence grain structure. EBM tends to produce less pronounced texture than L-PBF due to higher bulk temperatures.
- Post-traitement : HIP and specific heat treatments can help homogenize the microstructure to some extent, but significant anisotropy may remain.
Successfully navigating these challenges requires deep expertise in materials science, process physics, DfAM, and rigorous process control. Collaborating with an experienced AM service provider like Met3dp, equipped with reliable machinery, high-quality materials, and a team that understands the intricacies of printing superalloys for demanding applications like turbine blades, is key to mitigating risks and achieving repeatable, high-quality results.
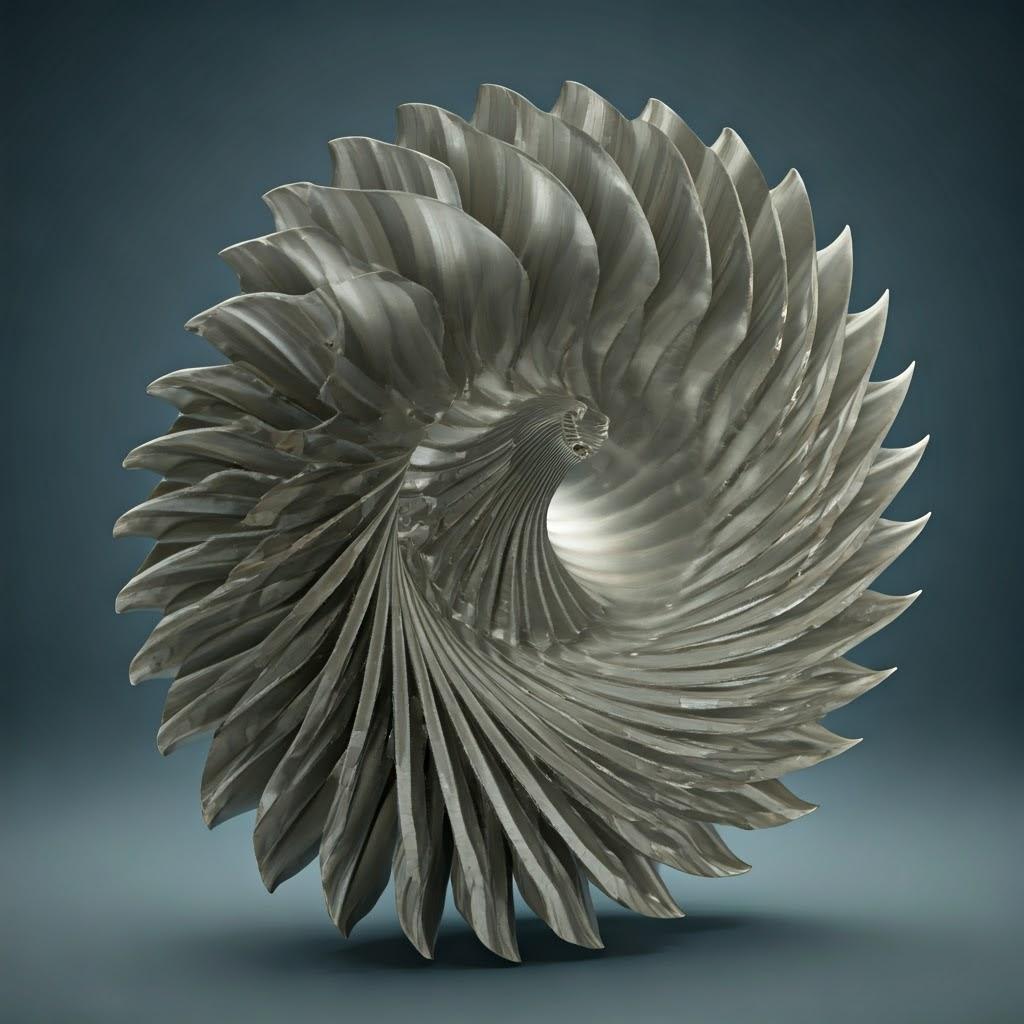
Selecting Your Partner: Choosing a Reliable Metal 3D Printing Supplier for Turbine Blades
The decision of which additive manufacturing service provider to partner with is particularly critical when producing high-stress, high-temperature components like turbine blades from superalloys. Not all providers possess the necessary expertise, equipment, and rigorous process control required for these demanding applications. Selecting the right partner is paramount to ensuring part quality, reliability, and adherence to stringent industry standards – a key consideration for B2B procurement in aerospace, power generation, and other critical sectors.
When evaluating potential metal AM suppliers for turbine blade projects, consider the following crucial criteria:
- Deep Material Expertise:
- Does the supplier have demonstrable experience printing the specific superalloy required (e.g., IN738LC, IN718, Rene 41)? This includes possessing validated, optimized process parameters.
- Do they understand the unique challenges associated with these materials (e.g., cracking susceptibility, heat treatment response)?
- What are their procedures for powder handling, storage, testing, and traceability to ensure material integrity?
- Appropriate Technology and Equipment:
- Do they operate the right type of AM technology (L-PBF, EBM) suitable for the material and application?
- Is their equipment well-maintained, calibrated, and capable of producing parts of the required size and complexity?
- Do they have sufficient machine capacity to meet potential production volume requirements and lead time expectations? A clear understanding of different méthodes d'impression and their trade-offs is beneficial.
- Robust Quality Management System (QMS) & Certifications:
- Is the supplier certified to relevant quality standards? ISO 9001 is a baseline; AS9100 is often required for aerospace components.
- Do they have documented procedures for process control, part inspection, traceability (from powder batch to final part), and handling non-conformances?
- Can they provide necessary documentation like Certificates of Conformance and material test reports?
- Comprehensive Post-Processing Capabilities:
- Turbine blades require extensive post-processing. Does the supplier offer these services in-house (preferred for control and lead time) or through a network of qualified and tightly managed partners?
- This includes stress relief, heat treatment (with precise atmosphere and temperature control), HIP, high-precision CNC machining, surface finishing, NDT (CT scanning, FPI), and coating preparation.
- Ingénierie et soutien du DfAM :
- Does the supplier have application engineers who can collaborate on design optimization (DfAM) to maximize printability, performance, and cost-effectiveness?
- Can they provide guidance on support strategies, orientation, and feature design limitations?
- Proven Track Record and Industry Experience:
- Can the supplier provide case studies or examples of successfully completed projects involving similar materials, complexities, and industry requirements?
- Are they experienced in serving the aerospace, power generation, or relevant industrial markets?
- Capacity, Scalability, and Project Management:
- Can they handle the required volume, from initial prototypes to potential low-to-medium series production?
- Do they have effective project management processes to ensure on-time delivery and clear communication?
- Confidentiality and Intellectual Property (IP) Protection:
- What measures are in place to protect sensitive design data and client IP? Ensure robust NDAs and secure data handling practices.
Choosing a supplier is about more than just price; it’s about finding a true partner with the technical depth and quality focus required for critical components. Companies like Met3dp, offering vertically integrated solutions encompassing advanced AM equipment, high-quality metal powders specifically designed for AM, and expert application development services, are positioned to be such partners. Our commitment to quality and understanding of the entire additive manufacturing workflow provides the reliability that industrial B2B customers demand.
Understanding Investment: Cost Factors and Lead Times for Industrial Turbine Blade Orders
Additive manufacturing offers significant advantages for turbine blade production, but understanding the associated costs and lead times is crucial for effective project planning and procurement. Unlike traditional high-volume manufacturing where tooling amortization heavily influences piece price, AM costs are driven by a different set of factors, and lead times depend heavily on the complete process chain, not just the print duration.
Key Cost Drivers for AM Superalloy Turbine Blades:
- Coût des matériaux :
- Nickel-based superalloys (IN738LC, IN718, Rene 41) are inherently expensive raw materials. The cost per kilogram of high-quality, gas-atomized powder suitable for AM is substantial.
- While AM minimizes waste compared to subtractive methods, the volume of material in the final part and required support structures directly impacts cost.
- Powder reusability/recycling practices of the supplier also influence effective material cost.
- Le temps des machines :
- This is often the largest single cost component. It’s primarily determined by:
- Part Volume: The overall size (bounding box) of the part determines how much space it occupies in the build chamber.
- Part Height: Dictates the number of layers required, directly impacting print duration.
- Complexity & Density: Highly complex internal structures or dense, solid sections require more scanning time per layer.
- Machine depreciation, maintenance, energy consumption, and inert gas usage are factored into the hourly machine rate.
- This is often the largest single cost component. It’s primarily determined by:
- Travail :
- Skilled labor is required for machine setup, build preparation (loading powder, slicing data), build monitoring, part removal, support removal, and extensive post-processing operations.
- Coûts de post-traitement :
- These steps add significantly to the total cost and cannot be overlooked:
- Heat Treatments (Stress Relief, Solution, Aging): Furnace time, energy, controlled atmospheres.
- Pressage isostatique à chaud (HIP) : A specialized, often expensive batch process required for densification and improved properties.
- Suppression du support : Can be labor-intensive, especially for complex internal supports.
- Usinage CNC : Required for critical tolerances; machining superalloys is slow and tool-intensive.
- Finition de la surface : Costs vary widely depending on the method (e.g., AFM, polishing) and required Ra value.
- CND et inspection : Costs associated with CT scanning, FPI, CMM, etc.
- These steps add significantly to the total cost and cannot be overlooked:
- Quality Assurance & Documentation:
- The overhead associated with maintaining a robust QMS, performing inspections, material testing (witness coupons), and generating certification paperwork contributes to the cost.
- Volume de commande :
- While AM doesn’t have the steep tooling cost amortization of casting, some economies of scale exist. Higher volumes allow for better nesting of parts within a build, potentially reducing machine time per part, and amortizing setup labor over more units. Suppliers may offer bulk order discounts. However, the cost curve for AM tends to be flatter than for traditional mass production methods.
Lead Time Considerations:
Lead time for a finished AM turbine blade is the sum of several stages:
- Quoting & Order Processing: Can range from days to weeks depending on complexity and supplier workload.
- Préparation de la construction : Data preparation, machine scheduling, setup (hours to days).
- Print Time: Highly variable based on size/complexity; can range from hours for small prototypes to many days for large, complex blades or full build plates.
- Cool Down & Part Removal: Heures.
- Post-traitement :This often dictates the overall lead time.
- Stress Relief/Heat Treatments: Days (including furnace cycles).
- HIP: Often requires batching parts and shipping to a specialized facility; can add 1-3 weeks.
- Machining/Finishing: Days to weeks depending on complexity and shop loading.
- NDT & Inspection: Days.
- Expédition: Variable based on location and method.
Typical Timelines (Highly Variable Estimates):
- Prototypes (basic post-processing): 1-4 semaines
- Fully Processed Production Parts (incl. HIP, full heat treat, machining, NDT): 6-12 weeks or potentially longer, depending heavily on the complexity and external process scheduling (like HIP).
For Procurement: It is essential to request detailed quotes that break down costs across printing and various post-processing stages. Similarly, request realistic lead time estimates that account for the entire workflow, not just the printing time. Clear communication and upfront planning with the supplier are key.
Frequently Asked Questions (FAQ) about Turbine Blade 3D Printing
As metal additive manufacturing becomes increasingly adopted for critical components like turbine blades, engineers and procurement managers often have questions about its capabilities, limitations, and comparison to traditional methods. Here are answers to some frequently asked questions:
Q1: How do the mechanical properties of 3D printed superalloy blades compare to cast or wrought equivalents? A : When using high-quality powders (like IN738LC, IN718, Rene 41) and optimized, certified processes including appropriate heat treatments and Hot Isostatic Pressing (HIP), the mechanical properties (tensile strength, yield strength, elongation, creep resistance, fatigue life) of AM superalloy parts can be highly comparable, and sometimes even superior to, investment cast equivalents. HIP is crucial for closing internal porosity, significantly improving fatigue properties. The fine-grained microstructure often achieved in AM can offer strength benefits. However, properties can exhibit some anisotropy (directionality) related to the build direction, which must be considered in design and qualification. Achieving properties equivalent to wrought materials is more challenging, as wrought alloys benefit from extensive thermo-mechanical processing, but AM parts often meet or exceed the requirements previously met by castings. Rigorous testing and qualification according to industry standards (e.g., AMS, MMPDS guidelines) are essential.
Q2: Is metal 3D printing cost-competitive with investment casting for turbine blades? A : The cost-competitiveness depends heavily on several factors:
- Volume : For very high-volume production runs (thousands of identical parts), investment casting often remains more economical due to well-amortized tooling costs and faster cycle times per part.
- La complexité : For blades with highly complex internal cooling channels or features difficult/impossible to cast, AM can be more cost-effective even at lower volumes by enabling designs that significantly improve performance or eliminate assembly steps.
- Délai de mise en œuvre: AM offers drastically reduced lead times for initial parts (no tooling needed), making it ideal for prototyping, rapid iteration, and urgent MRO needs. This speed can provide significant economic value.
- Legacy Parts: For older systems where casting tooling no longer exists, AM is often the only viable option for producing replacement parts.
- Total Cost of Ownership: Consider the entire lifecycle. If AM enables a lighter, more efficient, or longer-lasting blade design, the higher initial piece price might be offset by operational savings. In summary: AM excels in cost-competitiveness for low-to-medium volumes, high complexity, rapid development, and on-demand spares.
Q3: What is the typical size limitation for printing turbine blades? A : The maximum printable size is dictated by the build volume of the specific AM machine used. Current state-of-the-art L-PBF and EBM machines have build envelopes that can accommodate a wide range of turbine blades, including most blades found in aero-engines and many industrial gas turbines (IGTs). Build volumes can range roughly from 250x250x300 mm up to 800x400x500 mm or even larger in some specialized systems. While most blades fit within these envelopes, the very largest blades from the biggest utility-scale IGTs might still exceed current single-print capabilities and could require traditional manufacturing or potentially printing in sections and joining. Always check the specific machine capabilities of your chosen supplier.
Q4: How is material certification handled for AM turbine blades? A : Material certification for critical AM parts is a rigorous process involving:
- Powder Control: Strict control and traceability of powder batches, including chemistry verification, PSD analysis, and morphology checks. Re-use strategies must be validated.
- Contrôle des processus : Locking down validated process parameters (laser power, speed, etc.) and ensuring machine calibration and environmental control.
- Witness Coupons: Printing standardized test specimens (tensile bars, creep/fatigue samples) alongside the actual parts within the same build job.
- Mechanical Testing: Extensive testing of these coupons (after identical post-processing as the parts) to verify that mechanical properties meet the required material specifications (e.g., AMS standards).
- CND et inspection : Performing required NDT (e.g., CT scanning) and dimensional inspection on the final parts.
- Documentation : Providing a comprehensive Certificate of Conformance (CoC) that includes powder batch information, processing details (confirmation of adherence to qualified process), heat treat/HIP records, NDT results, and mechanical test reports from the witness coupons.
Q5: What is the expected lifespan or durability of a 3D printed turbine blade? A : The goal of using AM for turbine blades is to produce parts with a lifespan and durability that meet or exceed those of their traditionally manufactured counterparts designed for the same operating conditions. When produced using qualified materials, validated processes, comprehensive post-processing (especially HIP and correct heat treatments), and rigorous quality control, AM turbine blades are designed and expected to achieve the required service life. The ability to create optimized cooling channels and potentially superior microstructural features can, in some cases, even lead to enhanced durability or performance. However, like any critical component, extensive engine testing and certification are required to validate the lifespan in the specific application environment before widespread deployment.
Conclusion: The Future of Turbine Blade Supply Chains with Metal 3D Printing
The manufacturing of turbine blades stands as a testament to engineering precision, operating at the harsh confluence of extreme temperatures, high stresses, and demanding performance requirements. While traditional methods like casting and forging have long been the standard, metal additive manufacturing, powered by advanced superalloys like IN738LC, IN718, and Rene 41, represents a profound shift, offering capabilities previously unattainable.
As we’ve explored, metal 3D printing unlocks unparalleled liberté de conception, enabling the creation of intricate internal cooling geometries and topologically optimized structures that boost turbine efficiency and performance. It dramatically reduces lead times for prototypes and production parts, accelerating innovation cycles and enabling responsive MRO strategies. Furthermore, AM offers significant potential for improved material utilization and the creation of resilient, agile supply chains through digital inventory and on-demand production.
However, harnessing these benefits requires navigating the complexities of the technology. Success hinges on meticulous Conception pour la fabrication additive (DfAM), careful sélection des matériaux coupled with high-quality powder, precisely controlled printing processes, and critical post-processing steps like heat treatment, HIP, and machining to achieve the required properties and tolerances. Overcoming challenges like residual stress, potential cracking, and ensuring dimensional accuracy demands deep expertise and rigorous quality control.
For engineers and procurement managers in aerospace, power generation, and related industries, embracing metal AM for turbine blade production is becoming a strategic necessity. It requires careful planning, clear specification of requirements, and, crucially, selecting the right manufacturing partner.
Met3dp stands ready to be that partner. With our roots in providing industry-leading additive manufacturing solutions, encompassing both advanced 3D printing systems and the production of high-performance spherical metal powders via cutting-edge atomization techniques, we offer a comprehensive approach. Our expertise spans the entire AM workflow, ensuring quality and reliability from powder feedstock to finished component verification. We collaborate with organizations to implement 3D printing effectively, helping them transform their manufacturing capabilities and accelerate their journey towards digital production.
The integration of metal additive manufacturing into the production of critical components like turbine blades is not just a trend; it is the future unfolding. It promises more efficient, higher-performing turbines and more responsive, robust supply chains. By partnering with knowledgeable and capable suppliers like Met3dp, businesses can confidently leverage this transformative technology to gain a competitive edge and power the next generation of innovation in flight and energy. Contact Met3dp today to explore how our capabilities can fuel your organization’s additive manufacturing ambitions.
Partager sur
MET3DP Technology Co. est un fournisseur de premier plan de solutions de fabrication additive dont le siège se trouve à Qingdao, en Chine. Notre société est spécialisée dans les équipements d'impression 3D et les poudres métalliques de haute performance pour les applications industrielles.
Articles connexes
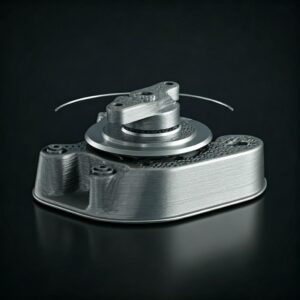
Supports imprimés en 3D pour les capteurs radar automobiles : Précision et performance
Lire la suite "À propos de Met3DP
Mise à jour récente
Notre produit
CONTACTEZ-NOUS
Vous avez des questions ? Envoyez-nous un message dès maintenant ! Nous répondrons à votre demande avec toute une équipe dès réception de votre message.
Obtenir les informations de Metal3DP
Brochure du produit
Obtenir les derniers produits et la liste des prix
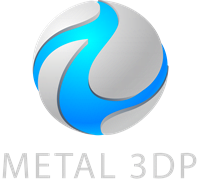
Poudres métalliques pour l'impression 3D et la fabrication additive
PRODUIT
cONTACT INFO
- Ville de Qingdao, Shandong, Chine
- [email protected]
- [email protected]
- +86 19116340731