Titanium Mounts for Aerospace Structures via 3D Printing
Obsah
Titanium Mounts for Aerospace Structures via 3D Printing: A Guide for Engineers and Procurement
The aerospace industry operates at the cutting edge of engineering, demanding components that offer exceptional performance, unwavering reliability, and minimal weight. Among the most critical yet often overlooked components are aerospace mounts. These vital pieces of hardware are the linchpin connecting various systems and structures within aircraft, spacecraft, satellites, and unmanned aerial vehicles (UAVs). As aerospace designs push towards greater efficiency and complexity, traditional manufacturing methods for these crucial parts are increasingly encountering limitations. This is where the transformative power of metal additive manufacturing (AM), commonly known as metal 3D printing, combined with high-performance materials like titanium alloys, is revolutionizing component design and production. For engineers seeking design innovation and procurement managers aiming for optimized supply chains and reduced lifecycle costs, understanding the potential of 3D printed titanium aerospace mounts is paramount.
Introduction: The Critical Role of Aerospace Mounts and the Rise of Titanium Additive Manufacturing
What Are Aerospace Mounts? The Unsung Heroes of Flight
Aerospace mounts, in their diverse forms, serve fundamental roles in ensuring the structural integrity, stability, and functionality of aerospace vehicles. They are essentially brackets, fixtures, or interfaces designed to securely attach, support, and position various components within an airframe or spacecraft structure. Their functions are multifaceted and critical:
- Structural Load Bearing: Mounts often transfer significant static and dynamic loads between components, such as engines and wing structures, landing gear and fuselage, or heavy equipment and internal frameworks. They must withstand immense forces during takeoff, landing, maneuvering (high G-forces), and turbulent conditions.
- Vibration Damping and Isolation: Aerospace environments are subject to intense vibrations from engines, aerodynamic forces, and machinery. Mounts are frequently designed to absorb or isolate these vibrations, protecting sensitive equipment (like avionics, sensors, cameras) and preventing fatigue failure in surrounding structures.
- Precise Positioning and Alignment: Many components, particularly sensors, guidance systems, and communication arrays, require precise and stable positioning. Mounts ensure these components maintain their required orientation and alignment under operational stresses.
- Tepelný management: In some applications, mounts can play a role in heat dissipation or provide thermal breaks between components operating at different temperatures.
- Securing Systems: They hold vital systems in place, including hydraulic lines, electrical harnesses, fuel system components, and environmental control systems.
Given these demanding roles, the failure of a single mount can have catastrophic consequences. Therefore, their design, material selection, and manufacturing process are subject to the highest standards of quality and reliability.
Traditional Manufacturing Challenges: The Constraints of the Past
Historically, aerospace mounts have been produced using conventional manufacturing techniques, primarily:
- Subtractive Machining: CNC machining parts from solid blocks (billets) of aerospace-grade metals like aluminum or titanium. While capable of high precision, this method suffers from:
- High Material Waste: Especially with complex geometries, a significant portion (often 80-95%) of the expensive raw material block is machined away, leading to a poor “buy-to-fly” ratio.
- Design Limitations: Certain complex shapes, internal features, or highly optimized structures can be difficult or impossible to machine efficiently or economically.
- Tooling and Setup Costs: Complex fixtures may be required, adding to cost and lead time.
- Obsazení: Pouring molten metal into molds. While suitable for certain shapes, casting can have limitations in terms of achievable tolerances, material properties (potential for porosity), and the need for significant post-machining. Creating the initial molds also involves time and cost.
- Fabrication/Assembly: Welding or fastening multiple simpler pieces together. This introduces potential failure points at joints, adds weight due to fasteners, and increases assembly time and complexity.
These traditional methods often result in longer lead times, particularly for complex or low-volume parts, hinder the implementation of highly optimized lightweight designs, and contribute significantly to material waste, a major cost driver in aerospace manufacturing. Procurement managers often face challenges sourcing these components from reliable dodavatelé leteckých komponentů who can meet demanding schedules and cost targets using these older techniques.
The Advent of Metal Additive Manufacturing in Aerospace
Metal Additive Manufacturing represents a paradigm shift. Instead of removing material, AM builds parts layer by layer directly from a 3D CAD model using high-energy sources like lasers or electron beams to fuse metal powder particles. This approach fundamentally overcomes many limitations of traditional methods, offering aerospace engineers and manufacturers unprecedented possibilities. Processes like Laser Powder Bed Fusion (LPBF, also known as Selective Laser Melting or SLM) and Electron Beam Melting (EBM) are increasingly being adopted for producing flight-critical components. The ability to create complex, lightweight, and high-performance parts directly from digital designs is driving significant innovation across the sector. Companies seeking to leverage this technology often look for an experienced kov 3D tisk dodavatel with a deep understanding of aerospace requirements.
Titanium: The Material of Choice for Demanding Applications
Titanium alloys, particularly Ti-6Al-4V (Grade 5) and its higher purity variant Ti-6Al-4V ELI (Grade 23), have become benchmark materials in aerospace due to their exceptional combination of properties:
- Vysoký poměr pevnosti k hmotnosti: Titanium offers strength comparable to many steels but at significantly lower density, making it ideal for weight-critical aerospace applications.
- Vynikající odolnost proti korozi: Titanium forms a stable, passive oxide layer, providing outstanding resistance to corrosion from jet fuel, hydraulic fluids, saltwater, and atmospheric conditions.
- Good High-Temperature Performance: It retains strength at moderately elevated temperatures found in engine compartments and airframes.
- Odolnost proti únavě: Crucial for components subjected to cyclic loading during flight operations.
The Synergy: AM and Titanium for Aerospace Mounts
Combining the design freedom of additive manufacturing with the superior properties of titanium alloys creates a powerful synergy for producing next-generation aerospace mounts. This combination enables:
- Optimized Designs: Creation of topologically optimized mounts that place material only where needed for load bearing, drastically reducing weight.
- Konsolidace částí: Designing single, complex mounts that replace assemblies of multiple simpler parts, reducing fasteners, assembly time, and potential failure points.
- Rychlé prototypování a iterace: Quickly producing and testing design variations to arrive at the optimal solution much faster than with traditional tooling-based methods.
- Snížení množství materiálového odpadu: AM processes like LPBF and EBM are near-net-shape, using only the material required for the part and support structures, significantly improving the buy-to-fly ratio compared to machining.
This technological advancement allows companies like Met3dp, with expertise in both advanced 3D tisk z kovu systems like their Selective Electron Beam Melting (SEBM) printers and high-performance metal powder production, to offer comprehensive solutions for manufacturing complex, high-value aerospace components.
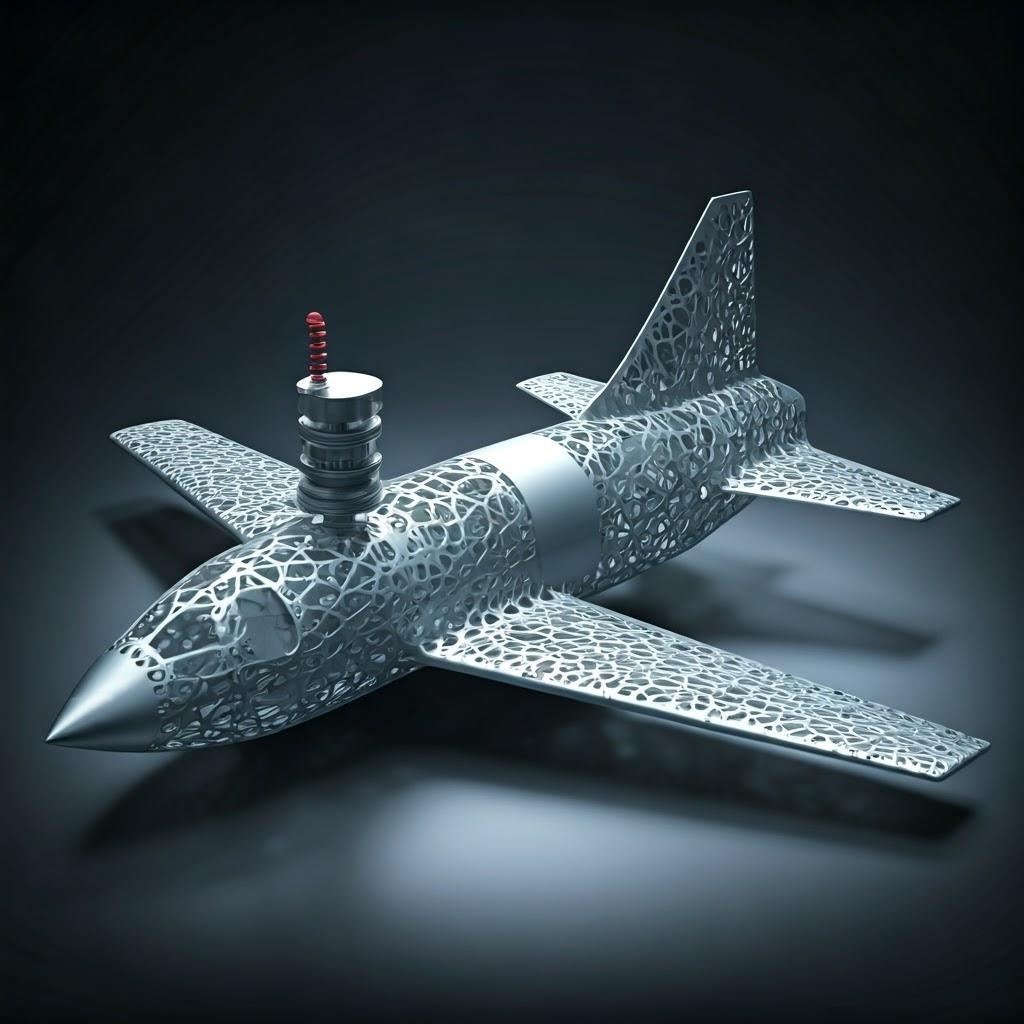
What Are 3D Printed Titanium Aerospace Mounts Used For? Applications and Functions
The versatility of metal additive manufacturing combined with the robust properties of titanium alloys like Ti-6Al-4V allows for the production of a wide array of aerospace mounts tailored to specific, demanding applications. These components are no longer limited by the constraints of traditional machining or casting, enabling optimized performance across various aerospace platforms. Procurement managers looking for a reliable titanium component supplier nebo aerospace bracket manufacturing partner need to understand the breadth of applications where 3D printed titanium mounts excel.
Detailed Breakdown of Mount Types:
While “mount” is a general term, 3D printing allows for highly specialized designs within this category:
- Engine Mounts and Pylon Brackets: These are highly critical structural components that attach engines to the aircraft wing or fuselage. They must handle extreme loads, high temperatures, and significant vibrations. AM allows for complex, weight-optimized designs that maintain structural integrity under immense stress. Topology optimization is frequently employed here to create organic-looking shapes that efficiently transfer load paths.
- Equipment Mounts and Racks (Avionics Trays): Securely housing sensitive and often heavy avionics equipment, control units, power distribution systems, and instrumentation. These mounts often require vibration isolation features, considerations for thermal management (heat dissipation), and precise positioning. AM enables the integration of complex internal features, snap-fits, or cable routing channels directly into the mount structure.
- Structural Brackets and Nodes: Connecting different parts of the airframe, such as wing ribs to spars, fuselage sections, or bulkhead reinforcements. These often have complex geometries dictated by the surrounding structures and load requirements. AM facilitates the creation of bespoke, lightweight nodes that can replace heavier, multi-part assemblies.
- Sensor and Antenna Mounts: Providing stable and precisely aligned platforms for critical sensors (e.g., air data sensors, navigation sensors, optical payloads) and communication antennas. These mounts must often be designed for specific aerodynamic profiles or thermal stability requirements. The ability to create custom shapes with AM ensures optimal placement and performance.
- Hydraulic, Fuel, and Electrical Line Mounts (Clamps/Brackets): Securing the vast network of lines and harnesses throughout an aircraft or spacecraft. While seemingly simple, these mounts are numerous and contribute to overall weight. AM allows for the design of highly optimized, lightweight clamps, potentially consolidating multiple line supports into a single printed component.
- Actuator Mounts: Supporting linear or rotary actuators used for flight control surfaces, landing gear deployment, or other mechanical systems. These must withstand significant operational loads and maintain precise alignment.
- Interior Mounts: Brackets for securing cabin elements, cargo restraints, or other internal fittings where weight savings are still beneficial.
Specific Aerospace Platforms and Applications:
3D printed titanium mounts are finding applications across the entire spectrum of aerospace and defense:
- Commercial Aircraft: Engine components, structural airframe brackets, cabin interior fittings, door mechanism components. The drive for fuel efficiency makes weight reduction paramount.
- Military Aircraft & Fighter Jets: High-performance structural parts, weapons system mounts, sensor pods, components requiring high strength and fatigue resistance under extreme G-loads and vibrations. Faster turnaround for replacement parts or upgrades is also a key benefit.
- Satelity a kosmické lodě: Structural nodes, antenna supports, brackets for sensitive optical instruments, propellant tank mounts, waveguide mounts. Weight reduction is absolutely critical for reducing launch costs. Materials like Ti-6Al-4V ELI are favored for their cryogenic performance. Finding suppliers for satellite component procurement who understand AM is increasingly important.
- Unmanned Aerial Vehicles (UAVs / Drones): Engine/motor mounts, landing gear components, sensor and payload integration brackets, structural frame elements. AM enables rapid development cycles and highly customized, lightweight designs essential for maximizing flight endurance and payload capacity for UAV structural parts.
- Helicopters: Gearbox mounts, rotor system components, vibration-sensitive equipment mounts. AM can help address the challenging vibration environment in rotorcraft.
- Space Launch Vehicles: Brackets and supports within rocket engine assemblies, propellant line mounts, structural connections capable of withstanding extreme launch forces and temperatures.
Key Functional Requirements Driving AM Adoption:
The demanding operational environment dictates stringent functional requirements for aerospace mounts, many of which are better met through AM:
Functional Requirement | How 3D Printed Titanium Mounts Excel | Relevance to B2B Buyers |
---|---|---|
High Strength-to-Weight | Titanium’s inherent property, further enhanced by topology optimization possible only with AM, minimizing material usage. | Reduced fuel consumption, increased payload capacity, lower launch costs. |
Load Bearing Capacity | Optimized designs ensure stress distribution along primary load paths. HIP post-processing ensures material integrity. | Enhanced structural reliability, meeting safety-critical performance standards. |
Tlumení vibrací | Complex geometries, including internal lattice structures, can be designed to absorb or shift vibration frequencies. | Improved lifespan of sensitive equipment, enhanced passenger comfort, reduced fatigue. |
Odolnost proti únavě | High-quality Ti-6Al-4V powder (like Met3dp’s) and post-processing (HIP, surface finishing) yield excellent fatigue properties. | Increased component lifespan, reduced maintenance intervals, improved safety. |
Tepelná stabilita | Titanium maintains properties over a reasonable temperature range. AM allows integration of cooling channels if needed. | Reliable performance in varying thermal environments (e.g., near engines). |
Odolnost proti korozi | Intrinsic property of titanium ensures longevity in harsh aerospace environments (humidity, fluids, salt spray). | Reduced maintenance, longer part life, suitability for diverse operating conditions. |
Složité geometrie | AM removes traditional manufacturing constraints, enabling highly complex, integrated, and organic shapes tailored to specific needs. | Optimal fit and function, part consolidation potential, design innovation. |
Konsolidace částí | Single AM part can replace an assembly of multiple parts, fasteners, and joints. | Reduced part count, weight savings, simplified assembly, fewer failure points. |
Rapid Availability (MRO) | Potential for on-demand printing of spares or replacement parts, reducing inventory needs and AOG (Aircraft on Ground) time. | Improved operational readiness, reduced warehousing costs, faster repairs. |
Export do archů
Beyond Aerospace:
The advantages driving the adoption of 3D printed titanium mounts in aerospace also make them attractive for other high-performance industries requiring lightweight, strong, and corrosion-resistant components, such as:
- High-Performance Automotive / Motorsport
- Obranné a vojenské vybavení
- Námořní aplikace
- Medical Implants (using biocompatible grades)
- Industrial Robotics and Automation
Engineers and procurement specialists in these fields can learn valuable lessons from the aerospace sector’s adoption of this technology. Partnering with a knowledgeable metal additive manufacturing distributor or service provider like Met3dp can facilitate the transfer of this technology to new applications.
Why Use Metal 3D Printing for Aerospace Mounts? Key Advantages Over Conventional Methods
The decision to shift from tried-and-tested conventional manufacturing methods to metal additive manufacturing for critical components like aerospace mounts isn’t taken lightly. It’s driven by a compelling set of advantages that address key challenges in aerospace design, production, and operation. For engineers pushing design boundaries and procurement teams focused on total cost of ownership and supply chain efficiency, AM offers tangible benefits that are hard to ignore. Let’s delve deeper into why metal 3D printing is becoming the preferred method for producing high-performance titanium aerospace mounts.
1. Unprecedented Design Freedom & Complexity:
This is arguably the most transformative advantage of AM. Traditional methods are inherently subtractive or formative, limiting the geometric possibilities. AM, being additive, liberates designers:
- Optimalizace topologie: Sophisticated software algorithms can analyze load paths and stresses within a component’s design space, removing material where it’s not needed and adding it only where required for structural integrity. This results in highly organic, efficient shapes that are often impossible or prohibitively expensive to machine. For mounts, this means achieving the required strength and stiffness with minimal mass.
- Mřížové struktury: AM enables the creation of internal lattice or cellular structures within solid parts. These lattices can significantly reduce weight while maintaining structural support, absorb energy/vibration, or facilitate fluid flow if designed as channels. Imagine a mount with a dense outer skin for load transfer but a lightweight internal lattice core.
- Konsolidace částí: Complex assemblies previously made from multiple individual parts, requiring fasteners, welding, or bonding, can often be redesigned and printed as a single, monolithic component. A mount integrated with its fixture points or incorporating features from adjacent parts eliminates assembly labor, removes potential failure points at joints, reduces part count (simplifying inventory and logistics for wholesale aerospace parts management), and often leads to further weight savings.
- Conformal Cooling/Heating Channels: If a mount supports heat-generating equipment or requires thermal stability, AM allows internal channels that conform precisely to the part’s shape to be integrated directly during the build process, enabling more efficient thermal management than drilled channels.
- Internal Features & Complex Geometries: Features like hidden voids, intricate internal passages, undercuts, and smoothly blending curvatures can be created with ease, allowing for highly optimized functional designs.
2. Significant Weight Reduction (Improved Buy-to-Fly Ratio):
Weight is a primary cost driver in aerospace. Every kilogram saved translates into potential fuel savings over the aircraft’s lifespan, increased payload capacity, or extended range/mission duration.
- Optimized Designs: As mentioned above, topology optimization and lattice structures enabled by AM directly lead to lighter parts (often 20-60% weight reduction compared to conventionally manufactured equivalents) without compromising performance.
- Near-Net Shape: AM processes build parts close to their final dimensions. While some post-machining of critical interfaces might be needed, the initial material usage is drastically lower than machining from a large billet. The “buy-to-fly” ratio (weight of raw material purchased vs. weight of the final part) is significantly improved. Reducing waste of expensive titanium is a major cost benefit appealing to manažeři nákupu v leteckém průmyslu.
Example Buy-to-Fly Ratio Comparison:
Výrobní metoda | Typical Buy-to-Fly Ratio (Titanium) | Implications |
---|---|---|
Tradiční CNC obrábění | 10:1 to 20:1 (or higher) | 90-95% material waste, high raw material cost. |
Aditivní výroba kovů | 1.5:1 to 3:1 | Minimal material waste, significant cost savings on raw material. |
Export do archů
3. Material Efficiency and Sustainability:
Closely linked to weight reduction is the efficient use of materials.
- Snížení množství odpadu: AM uses only the material needed for the part and its supports (which can often be minimized through smart design and orientation). This dramatically cuts down on the amount of high-value titanium that ends up as machining chips, reducing environmental impact and raw material costs.
- Powder Reusability: Unfused powder within the build chamber can typically be recovered, sieved, and blended with virgin powder for reuse in subsequent builds (following strict quality control protocols), further improving material utilization. Companies like Met3dp, manufacturing their own powders, have optimized processes for powder lifecycle management.
4. Lead Time Reduction and Faster Iteration:
In the fast-paced aerospace industry, speed matters – both for development and for MRO (Maintenance, Repair, Overhaul).
- Rychlé prototypování: AM allows engineers to produce physical prototypes of mount designs in days rather than weeks or months associated with traditional tooling and machining setups. This enables faster design validation, functional testing, and iteration cycles.
- Tooling Elimination: AM typically requires no part-specific tooling (molds, dies, complex fixtures). This eliminates the significant time and cost associated with tool design, fabrication, and modification. Changes can be made directly in the CAD model and a new part printed quickly.
- Výroba na vyžádání: For spares or low-volume production runs, AM offers the potential for on-demand manufacturing. This reduces the need for large inventories of physical parts, potentially shortening AOG (Aircraft on Ground) times by printing replacement mounts as needed. This agility is crucial for aerospace MRO solutions.
- Digital Inventory: Designs exist as digital files, enabling distributed manufacturing closer to the point of need, potentially simplifying global supply chains.
5. Supply Chain Resilience and Agility:
Reliance on complex, multi-step traditional supply chains can be a vulnerability. AM offers alternatives:
- Reduced Supplier Dependencies: Part consolidation can reduce the number of individual component suppliers needed.
- Distributed Manufacturing: Digital part files can be sent electronically to certified metal AM service bureaus or internal facilities globally for local production, reducing shipping times and logistical hurdles.
- Faster Response to Design Changes: Engineering changes can be implemented much more quickly without the need for retooling.
6. Enhanced Performance and Functionality:
Beyond just replicating existing designs, AM can actually enhance component performance:
- Tailored Microstructures: Depending on the AM process (e.g., LPBF vs. EBM) and parameters used, different microstructures can be achieved, potentially influencing strength, fatigue life, and other properties. Post-processing like HIP is crucial for optimizing these properties.
- Integrated Functionality: As discussed under Design Freedom, features like vibration damping lattices or conformal cooling channels can be built directly into the mount, improving overall system performance.
- Biomimicry: AM allows designers to mimic efficient structures found in nature (like bone structures) for optimal strength-to-weight ratios.
In summary, metal additive manufacturing provides a compelling value proposition for producing titanium aerospace mounts, offering advantages in design flexibility, weight reduction, material efficiency, speed, supply chain agility, and potential performance enhancements that traditional methods cannot match. Leveraging advanced platforms like Met3dp’s SEBM printers and high-quality materials is key to unlocking these benefits reliably.
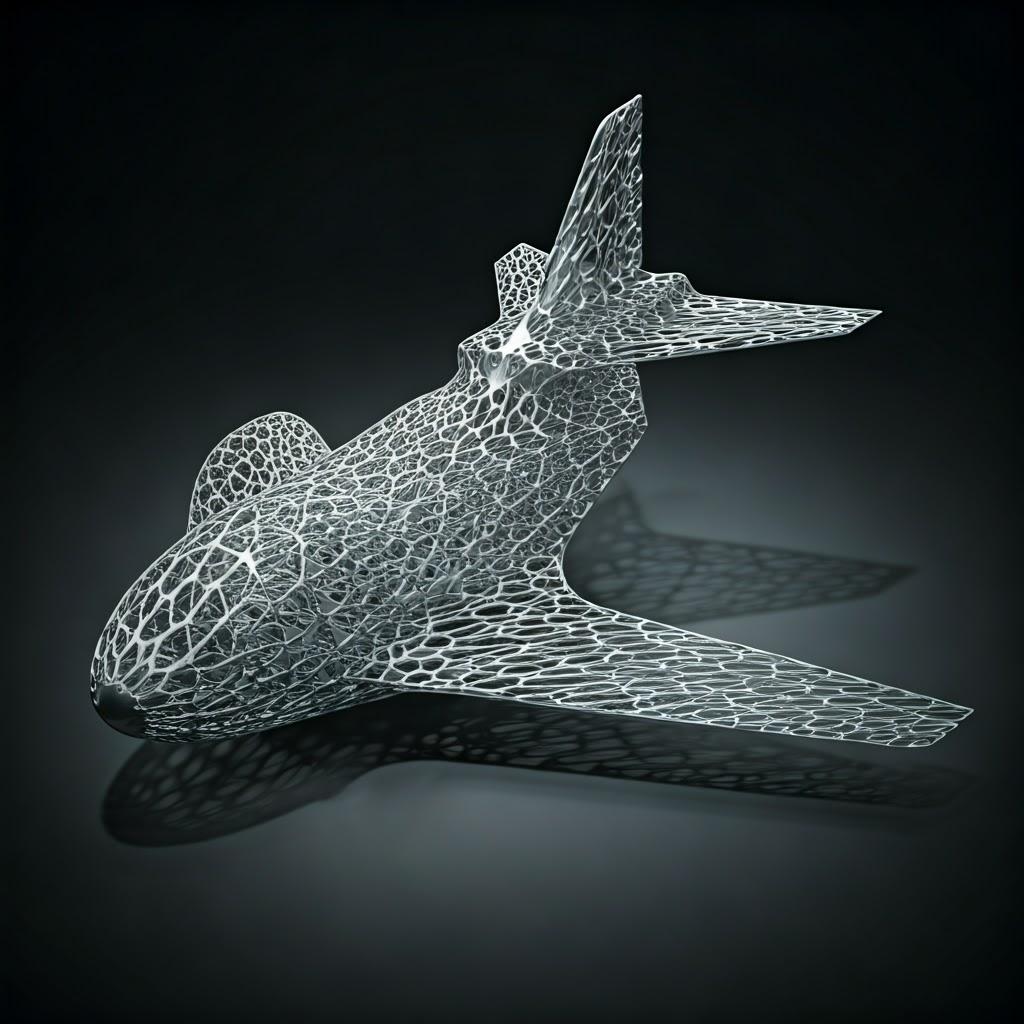
Recommended Materials for 3D Printed Aerospace Mounts: Ti-6Al-4V & Ti-6Al-4V ELI Deep Dive
Selecting the right material is fundamental to the success of any aerospace component, especially load-bearing mounts. While various alloys can be processed using metal additive manufacturing, Titanium alloys, specifically Ti-6Al-4V (Grade 5) and Ti-6Al-4V ELI (Grade 23), stand out as the workhorse materials for 3D printed aerospace mounts due to their exceptional balance of properties. Understanding their nuances is crucial for both design engineers specifying the material and procurement managers sourcing it from a qualified dodavatel titanového prášku or AM service provider.
Ti-6Al-4V (Grade 5): The Aerospace Standard
Ti-6Al-4V, often referred to simply as “Ti64” or Grade 5 titanium, is the most widely used titanium alloy across all industries, particularly aerospace. Its popularity stems from a highly desirable combination of characteristics achievable through AM:
- Vysoký poměr pevnosti k hmotnosti: This is its hallmark property. It offers strength comparable to many steel alloys at roughly 56% of the density. This directly translates to lighter components without sacrificing mechanical performance.
- Vynikající odolnost proti korozi: Forms a highly stable, tenacious passive oxide layer (TiO2) that protects it from corrosion in a wide range of aggressive environments, including jet fuel, hydraulic fluids, de-icing salts, and marine atmospheres.
- Good Elevated Temperature Capability: It can operate continuously at temperatures up to approximately 315°C (600°F) and withstand intermittent exposure to higher temperatures, making it suitable for applications near engines or in hot sections of the airframe.
- Weldability & Fabricability: Although we are focused on AM, its baseline weldability indicates good fusion characteristics essential for powder bed fusion processes.
- Moderate Cost (for Titanium): While more expensive than aluminum or steel, it is the most common and generally most cost-effective titanium alloy.
Ti-6Al-4V ELI (Grade 23): Enhanced Toughness and Purity
Ti-6Al-4V ELI (Extra Low Interstitials) is a higher-purity version of Grade 5. The key difference lies in the strictly controlled lower limits for interstitial elements, primarily oxygen and iron.
- Improved Ductility and Fracture Toughness: The reduction in interstitial elements significantly enhances the alloy’s ductility (ability to deform without fracturing) and fracture toughness (resistance to crack propagation), particularly at cryogenic temperatures.
- Superior Damage Tolerance: This makes Grade 23 the preferred choice for critical structural components where fail-safe or damage-tolerant design philosophies are employed, or where operation in very cold environments (like space or high-altitude flight) is expected.
- Zvýšená biokompatibilita: The higher purity also makes Grade 23 a common choice for medical implants, although this is less relevant for typical aerospace mounts.
- Slightly Lower Strength: The improved toughness comes at the cost of slightly lower tensile and yield strength compared to standard Grade 5.
Comparative Properties (Typical Values for AM parts after Stress Relief/HIP):
Vlastnictví | Ti-6Al-4V (Grade 5) AM | Ti-6Al-4V ELI (Grade 23) AM | Jednotka | Significance for Aerospace Mounts |
---|---|---|---|---|
Hustota | ~4.43 | ~4.43 | g/cm³ | Fundamental to high strength-to-weight ratio. |
Pevnost v tahu (UTS) | 950 – 1150 | 860 – 1000 | MPa | Maximum stress the material can withstand before fracture. |
Mez kluzu (posun 0,2%) | 850 – 1050 | 790 – 930 | MPa | Stress at which permanent deformation begins; critical for design limits. |
Prodloužení po přetržení | 8 – 15 | 10 – 18 | % | Measure of ductility; higher value in ELI indicates better toughness. |
Fracture Toughness (K<sub>IC</sub>) | 55 – 70 | 70 – 95 | MPa√m | Resistance to crack propagation; significantly higher in ELI, crucial for damage tolerance. |
Fatigue Strength (Rotating Beam) | ~500 – 600 | ~500 – 600 | MPa | Resistance to failure under cyclic loading; vital for longevity in vibrating environments. |
Max Operating Temperature | ~315 | ~315 | °C | Suitable for many airframe and some engine-adjacent applications. |
Key Interstitial Limits | O < 0.20%, N < 0.05%, Fe < 0.30% | O < 0.13%, N < 0.03%, Fe < 0.25% | Hmotnost % | Lower interstitials in ELI are the primary differentiator, improving toughness. |
Relevant AMS Standards (Typical) | AMS 4911, AMS 6931 (LPBF), AMS 7001 (EBM) | AMS 4907, AMS 6930 (LPBF), AMS 7000 (EBM) | – | Certifications often require adherence to specific aerospace material specifications (AMS/ASTM). |
Export do archů
(Note: Specific properties depend heavily on AM process parameters, build orientation, and post-processing (especially heat treatment/HIP). These are representative values.)
Why These Alloys Are Ideal for Aerospace Mounts:
The combination of high strength, low density, excellent corrosion resistance, good fatigue life, and adequate temperature resistance makes both Ti-6Al-4V and Ti-6Al-4V ELI exceptionally well-suited for the demanding requirements of aerospace mounts.
- 5. třída is often sufficient for a wide range of mounts where maximum strength and cost-effectiveness are priorities.
- Třída 23 is selected for highly critical mounts requiring superior damage tolerance, applications involving cryogenic temperatures (space, high altitude), or where specific fracture mechanics requirements must be met.
The Critical Importance of High-Quality Metal Powder:
The success of producing reliable, high-performance 3D printed titanium mounts begins with the quality of the raw material: the metal powder. Unlike traditional metallurgy where bulk material properties are the starting point, in AM, the powder characteristics directly influence the quality of the final part layer by layer. Key powder characteristics include:
- Sféricita: Highly spherical powder particles flow evenly and pack densely in the powder bed. This ensures consistent layer deposition and uniform melting, minimizing the risk of voids or porosity in the final part. Irregular or satellite-bound particles can lead to poor flowability and packing density.
- Tekutost: Directly related to sphericity and particle size distribution, good flowability ensures the recoater blade can spread smooth, uniform layers of powder across the build platform, crucial for dimensional accuracy and part quality.
- Čistota: Contaminants (like excessive oxygen, nitrogen, carbon, or foreign particles) can severely degrade the mechanical properties (especially ductility and fatigue life) of titanium alloys. Strict control over the powder manufacturing process is essential to maintain high purity levels, particularly the low interstitial content required for ELI grades.
- Distribuce velikosti částic (PSD): The range and distribution of particle sizes affect powder bed density, flowability, and the achievable resolution and surface finish of the printed part. Different AM machines (LPBF vs. EBM) are optimized for specific PSD ranges. Consistency in PSD from batch to batch is vital for process repeatability.
Met3dp: Ensuring Powder Excellence for Critical Applications
Recognizing that powder quality is paramount, companies like Met3dp have invested heavily in state-of-the-art powder production technologies. Met3dp employs industry-leading methods such as:
- Vacuum Induction Melting Gas Atomization (VIGA): This process melts the pre-alloyed titanium feedstock in a vacuum or inert atmosphere and then disintegrates the molten metal stream using high-pressure inert gas jets (Argon or Nitrogen). This technique is renowned for producing highly spherical powders with good purity and controlled PSD, ideal for LPBF processes. Met3dp’s equipment utilizes unique nozzle and gas flow designs to optimize sphericity and flowability.
- Proces plazmové rotující elektrody (PREP): In PREP, a rapidly rotating electrode bar made of the target alloy is melted at its tip by a plasma torch. Centrifugal force then flings off molten droplets, which solidify in flight into highly spherical particles within an inert atmosphere. PREP is known for producing exceptionally clean and spherical powders with minimal satellites and internal porosity, highly desirable for critical applications and often favored for EBM processes.
By controlling the entire powder manufacturing process in-house, from raw material selection to atomization and classification, Met3dp ensures the consistent supply of high-purity, highly spherical Ti-6Al-4V and Ti-6Al-4V ELI powders optimized for the demanding requirements of aerospace additive manufacturing. Their portfolio also includes innovative alloys beyond the standards, offering further possibilities. This commitment to material quality makes Met3dp a reliable partner and high-performance metal powder supplier for companies seeking to produce flight-worthy 3D printed components. Procurement teams can have confidence in the traceability and quality control inherent in Met3dp’s powder production.
Design Considerations for Additive Manufacturing of Aerospace Mounts
Transitioning from designing for traditional manufacturing (like machining or casting) to designing for additive manufacturing (DfAM) requires a shift in mindset. Instead of being constrained by tool access or mold limitations, engineers can leverage the layer-by-layer freedom of AM to create highly optimized, complex, and lightweight aerospace mounts. However, successful AM also comes with its own set of design rules and considerations related to support structures, thermal management, and process capabilities. Embracing DfAM principles is crucial to fully unlock the potential benefits – weight reduction, part consolidation, and enhanced performance – that make 3D printed titanium mounts so compelling for the aerospace industry. Engineers collaborating with an experienced DfAM aerospace service provider can significantly shorten the learning curve.
Leveraging DfAM (Design for Additive Manufacturing) Principles:
DfAM isn’t just about making existing designs printable; it’s about fundamentally rethinking the design approach to maximize the advantages of the AM process while mitigating its constraints. Key DfAM principles for aerospace mounts include:
- Function-Driven Geometry: Focus on the functional requirements (load paths, stiffness, vibration modes, interfaces) and let these drive the geometry, rather than conforming to shapes easily produced by older methods.
- Complexity is (Almost) Free: Unlike machining, where complexity adds significant cost, adding intricate features, internal channels, or complex curvatures in AM has minimal impact on production cost, which is primarily driven by volume and height. Leverage this to integrate functionality.
- Design for Minimal Supports: While supports are often necessary, they add material cost, print time, and post-processing effort, and can mar surfaces. Design parts with self-supporting angles where possible and consider build orientation carefully.
- Design for Post-Processing: Ensure critical features are accessible for support removal, inspection, and any required machining or surface finishing. Consider adding sacrificial material (machining allowance) on critical surfaces.
- Thermal Management Considerations: Think about how heat will build up and dissipate during the printing process. Avoid very thick sections adjacent to very thin ones without smooth transitions, as this can exacerbate thermal stresses and warping.
Topology Optimization: Sculpting with Stress:
Topology optimization is a cornerstone of DfAM for structural parts like aerospace mounts. It’s a computational method that optimizes material layout within a defined geometric design space based on given load conditions, boundary constraints, and performance objectives (e.g., maximize stiffness, minimize mass).
- The Process:
- Define Design Space: Specify the maximum allowable volume the part can occupy.
- Define Loads & Constraints: Apply realistic operational load cases (tension, compression, shear, vibration) and define fixed points or “keep-out” zones where material cannot exist (e.g., bolt holes, interface surfaces).
- Set Objectives: Define the goal – typically minimizing mass while meeting specific stiffness or stress limits.
- Manufacturing Constraints: Input limitations of the AM process, such as minimum feature size or self-supporting angles, to ensure the resulting design is manufacturable.
- Run Optimization: The software iteratively removes material from low-stress areas, leaving behind an optimized load-bearing structure.
- The Result: Often organic, “bionic” looking structures that efficiently transfer loads with minimal material usage, leading to significant weight savings (often 20-50% or more).
- Software Tools: Common platforms include Altair Inspire, Ansys Discovery, Dassault Systèmes (SOLIDWORKS Simulation/CATIA Generative Design), nTopology, Autodesk Fusion 360.
- Úvahy: The raw output often needs some smoothing and refinement for manufacturability and fatigue considerations. It requires accurate load case definition for reliable results.
Support Structures: The Necessary Scaffolding:
In powder bed fusion processes (LPBF, EBM), overhangs and horizontal features require support structures beneath them to prevent collapse during the build and to anchor the part to the build plate, counteracting warping forces.
- Účel:
- Support down-facing surfaces and features below a critical self-supporting angle (typically <45° from horizontal).
- Act as heat sinks, drawing heat away from the melt pool, especially on overhangs.
- Secure the part to the build plate against thermal stresses and recoater forces.
- Typy: Can range from solid blocks to fine lattices, grids, or tree-like structures. The choice depends on the location, required strength, and ease of removal.
- Design Strategies:
- Minimize Need: Orient the part to maximize self-supporting surfaces. Use chamfers or fillets instead of sharp horizontal overhangs where possible.
- Optimize for Removal: Use support structures with small contact points (perforations, tapered connections) where they meet the part to ease break-off. Ensure physical access for tools if manual or machined removal is planned. Avoid supports in inaccessible internal channels unless absolutely necessary and designed for powder removal.
- Thermal Considerations: Denser supports may be needed in areas prone to overheating or warping.
- Dopad: Supports consume material and machine time, require significant post-processing labor for removal, and can affect the surface finish of the areas they touch. Efficient support strategies are key to cost-effective AM.
Orientation Strategy: Setting the Stage for Success:
How a part is oriented on the build plate profoundly impacts several aspects of the print:
- Doba výstavby: Primarily determined by the height (number of layers) in the Z-direction. Orienting the part flatter generally reduces build time but increases the cross-sectional area per layer and potentially the need for supports.
- Support Volume: Different orientations will result in varying amounts of required support structures. Minimizing supports is often a key driver for orientation choice.
- Kvalita povrchu: Surfaces angled relative to the build plate exhibit a “stair-stepping” effect due to the layered nature of AM. Vertical walls tend to have better finish than shallow angles. Downward-facing surfaces where supports attach will typically have lower surface quality after removal.
- Mechanical Properties (Anisotropy): Due to the directional nature of solidification and thermal gradients, AM parts can exhibit anisotropic properties (varying strength/ductility depending on the direction of testing relative to the build direction). This effect is process and material dependent but must be considered, especially for fatigue-critical parts. Critical load paths should ideally be aligned with the direction of optimal properties (often parallel to the build plate).
- Thermal Stress & Warping: Orientation affects how heat builds up and dissipates, influencing residual stress and the risk of warping. Orienting large flat surfaces parallel to the build plate can increase warping risk.
- Feature Resolution: The accuracy and definition of small features can vary slightly depending on their orientation relative to the layer lines.
Choosing the optimal orientation often involves balancing these competing factors based on the specific part geometry and requirements.
Wall Thickness & Feature Size:
AM processes have limits on the minimum size of features they can reliably produce:
- Minimum Wall Thickness: Typically around 0.4 – 1.0 mm, depending on the process (LPBF generally finer than EBM), machine, material, and wall height/aspect ratio. Very thin, tall walls are prone to distortion or failure during printing.
- Minimum Hole/Pin Diameter: Small holes (typically < 0.5 – 1.0 mm) may seal over during printing or be difficult to clear of powder. Horizontal holes often print slightly elliptical. Small pins may lack strength or definition.
- Self-Supporting Angles: Features angled more than ~45° from the horizontal plane can typically be built without supports. Angles shallower than this require support.
- Designing for Limits: Ensure critical features meet minimum size requirements. Consider designing features slightly larger if post-machining (e.g., drilling holes to final size) is planned.
Part Consolidation: Reducing Complexity:
One of the most powerful DfAM techniques is consolidating multiple components of an assembly into a single, monolithic printed part.
- Example: A complex aerospace mount assembly might consist of a main machined bracket, several smaller sheet metal stiffeners, and various fasteners (bolts, nuts, rivets). A DfAM approach could redesign this as a single, topologically optimized titanium part printed using AM.
- Výhody:
- Reduced Part Count: Simplifies bill of materials, inventory management, and supply chain logistics.
- Elimination of Joints/Fasteners: Reduces weight, removes potential failure points, eliminates assembly labor.
- Improved Structural Efficiency: Allows for smoother load transfer compared to bolted joints.
- Potential for Integrated Functionality: Incorporate features like cable clips or vibration damping structures directly.
Incorporating Internal Features:
AM’s layer-by-layer approach enables the easy creation of complex internal geometries:
- Lattices: Periodic (e.g., cubic, octet-truss) or stochastic (foam-like) lattices can be designed within solid volumes to reduce weight significantly while tailoring stiffness and energy absorption properties. Key consideration: ensuring internal powder can be removed.
- Internal Channels: Conformal channels for cooling fluids, hydraulics, or wiring can be integrated directly into the mount structure, protected from the external environment. Design must allow for powder evacuation.
By carefully considering these DfAM principles, engineers can design aerospace mounts that are not just manufacturable via AM, but are truly optimized for performance, weight, and cost-effectiveness within the aerospace context.
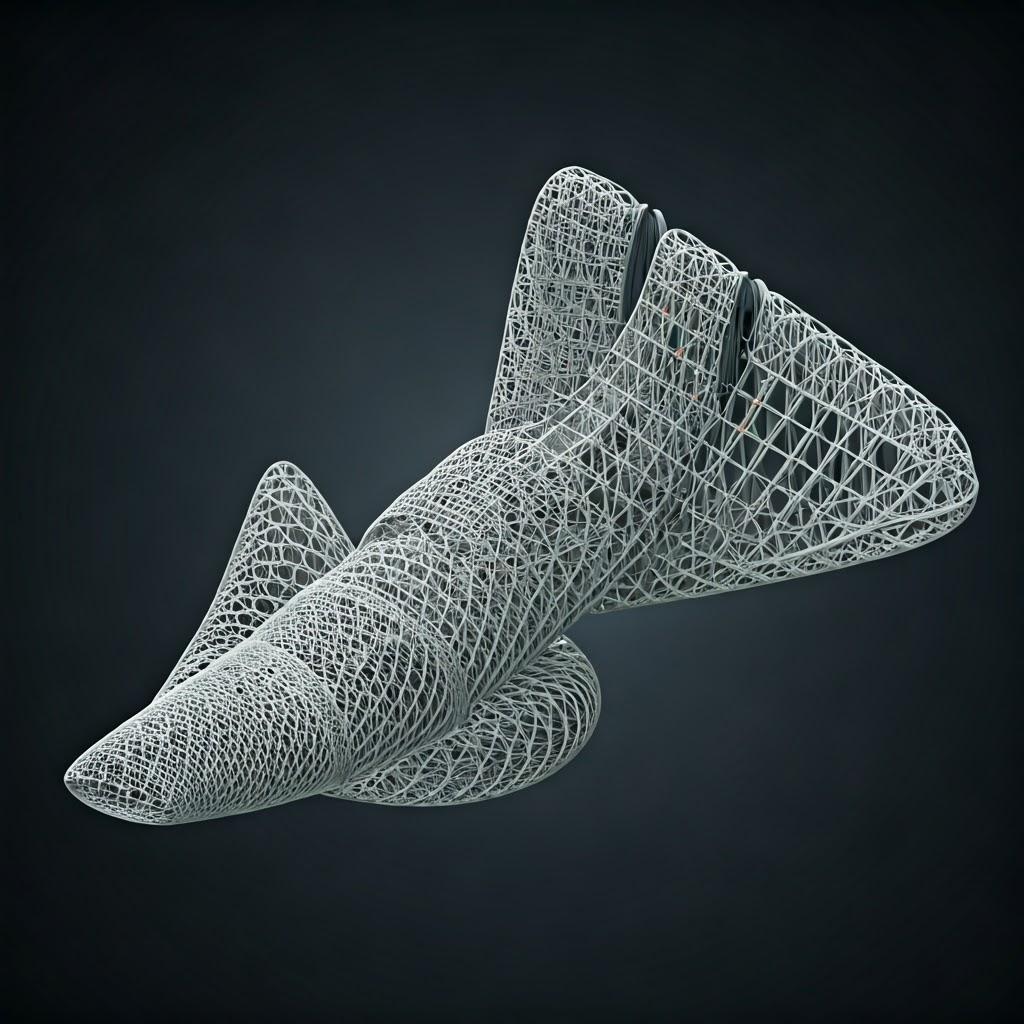
Tolerance, Surface Finish, and Dimensional Accuracy in 3D Printed Titanium Mounts
While additive manufacturing offers incredible design freedom, understanding the achievable levels of precision is critical for components like aerospace mounts, which often have strict requirements for fit, assembly, and function. Engineers and procurement managers must have realistic expectations regarding tolerances, surface finish, and overall dimensional accuracy of 3D printed titanium parts, and plan for necessary post-processing steps like machining to meet the tightest specifications. The choice of specific kovové metody 3D tisku like Laser Powder Bed Fusion (LPBF) or Electron Beam Melting (EBM) also influences these outcomes.
Achievable Tolerances with Metal AM:
Metal AM processes build parts layer by layer, and factors like thermal expansion/contraction, beam/laser spot size, powder characteristics, and machine calibration inherently limit the achievable “as-built” tolerances.
- General Ranges: Typical achievable tolerances for well-controlled LPBF or EBM processes producing titanium parts often fall within ±0.1 mm to ±0.3 mm for smaller dimensions, or ±0.1% to ±0.2% of the nominal dimension for larger features. This might correspond roughly to ISO 2768-m (medium) or sometimes -f (fine) tolerance classes for general dimensions.
- Factors Influencing Tolerances:
- AM proces: LPBF generally offers slightly better accuracy and finer feature resolution than EBM due to smaller layer thicknesses and finer powder/beam spot size. EBM’s higher processing temperature can reduce residual stress but may impact fine feature definition.
- Machine Calibration & Condition: Regular calibration and maintenance are crucial for accuracy.
- Part Size & Geometry: Larger parts or parts with significant thermal mass variations are more susceptible to distortion and tolerance deviations.
- Thermal Stresses: Uneven heating and cooling cause shrinkage and warping, which must be anticipated and compensated for (e.g., through simulation, support strategies, or design modifications).
- Orientace na stavbu: Affects how thermal stresses accumulate and how stair-stepping impacts angled surfaces.
- Kvalita prášku: Consistent particle size and morphology contribute to predictable melting and solidification.
- Následné zpracování: Stress relief heat treatments can cause minor dimensional changes. Support removal can affect surface accuracy.
- Meeting Tight Tolerances: For critical features requiring tolerances tighter than the general AM process capability (e.g., bearing bores, precise mating surfaces, alignment features), post-machining (CNC milling, turning, grinding) is almost always necessary. It’s crucial to include machining allowances in the DfAM phase for these features.
Surface Finish (Roughness): As-Built vs. Post-Processed:
The surface finish of AM parts is inherently rougher than machined surfaces due to the layer-by-layer fusion process and partially melted powder particles adhering to the surface.
- Drsnost povrchu (Ra) podle stavu:
- LPBF (SLM): Obvykle se pohybuje od 6 µm to 15 µm Ra, depending on parameters, orientation, and material. Vertical walls are generally smoother than angled or horizontal surfaces.
- EBM: Generally rougher than LPBF, typically ranging from 20 µm to 35 µm Ra due to larger powder particles and higher processing temperatures causing more sintering.
- Stair-Stepping Effect: Surfaces built at an angle to the build plate exhibit visible layer lines, contributing to roughness. The severity depends on the angle and layer thickness.
- Impact of Surface Finish: Rough surfaces can act as stress concentrators, negatively impacting fatigue life. They may also be unsuitable for sealing surfaces or aerodynamic applications.
- Improving Surface Finish (Post-Processing): Various methods can significantly improve the surface finish:
- Abrasive Blasting (Bead/Sand Blasting): Provides a uniform matte finish, typically 3-8 µm Ra. Good for removing loose powder and achieving cosmetic uniformity.
- Obrábění / vibrační úprava: Parts are tumbled with media, smoothing surfaces and edges. Can achieve 1-5 µm Ra. Best for batches of smaller, robust parts.
- CNC obrábění: Offers the best surface finish control, capable of achieving <1 µm Ra on specific features.
- Leštění (ruční nebo automatické): Can achieve very smooth, mirror-like finishes (<0.5 µm Ra) but is often labor-intensive.
- Electrochemical Polishing: Smooths surfaces electrochemically, effective on complex shapes but requires specific electrolytes.
Dimensional Accuracy and Verification:
Ensuring the final part meets all dimensional specifications is critical for aerospace components. This requires robust quality control and metrology.
- Řízení procesu: Maintaining tight control over AM process parameters (laser/beam power, speed, layer thickness, gas flow, temperature), using high-quality, consistent powder, and ensuring proper machine calibration are fundamental to achieving predictable dimensional outcomes. Companies like Met3dp emphasize the accuracy and reliability of their SEBM printers, built on rigorous process control.
- Metrology & Inspection:
- Coordinate Measuring Machines (CMMs): Provide high-accuracy point measurements for verifying critical dimensions, GD&T (Geometric Dimensioning and Tolerancing) features, and overall form tolerance.
- 3D Scanning (Laser/Structured Light): Capture dense point cloud data of the entire part surface, allowing comparison to the original CAD model (color map deviation analysis) and verification of complex geometries. Useful for identifying warping or distortion.
- Computed Tomography (CT) Scanning: Uses X-rays to create a 3D reconstruction of the part, allowing for non-destructive measurement of internal features, wall thicknesses, and detection of internal defects (porosity) inaccessible by other methods. Crucial for verifying complex internal channels or lattices designed using DfAM.
- Iterative Improvement: Measurement data can be fed back into the design and build preparation phase to apply compensation factors, improving the accuracy of subsequent prints.
Meeting Stringent Aerospace Requirements:
Aerospace standards demand high levels of precision and reliability. While AM provides the initial near-net shape, a combination of process control, DfAM (including machining allowances), carefully planned post-processing, and rigorous metrology is required to ensure 3D printed titanium mounts meet the demanding fit, form, and function requirements of flight-critical applications. Collaboration between design engineers, AM specialists, and quality assurance teams is essential throughout the process. Procurement managers should ensure potential dodavatelé aditivní výroby pro letecký průmysl have robust quality management systems (QMS) and metrology capabilities.
Post-Processing Requirements for 3D Printed Aerospace Mounts
A common misconception about metal additive manufacturing is that parts come out of the printer ready to use. In reality, particularly for demanding aerospace applications, the printing process is just one step in the manufacturing workflow. A series of essential post-processing steps are required to transform the as-built part into a functional, reliable, and flight-worthy aerospace mount. These steps are critical for relieving stress, removing supports, achieving required tolerances and surface finish, ensuring material integrity, and verifying quality. Understanding these requirements is vital for accurately estimating costs, lead times, and ensuring the final component meets stringent aerospace part qualification normy.
1. Stress Relief / Heat Treatment:
- Why it’s Critical: The rapid heating and cooling cycles inherent in powder bed fusion processes create significant residual stresses within the printed titanium part. These stresses can cause warping/distortion (especially after removal from the build plate), micro-cracking, and severely compromise the part’s mechanical performance, particularly fatigue life.
- The Process: Parts are typically subjected to a controlled thermal cycle in a vacuum or inert atmosphere furnace. Common treatments for Ti-6Al-4V include:
- Stress Relief Anneal: Heating to a specific temperature below the beta transus (e.g., 650-800°C for Ti-6Al-4V, soak time depends on thickness) followed by controlled cooling. This significantly reduces residual stresses while minimally affecting the microstructure and strength.
- Full Annealing: Heating to a higher temperature, sometimes above the beta transus, for longer times to achieve maximum ductility and stability, often at the expense of some strength.
- Solution Treating and Aging (STA): Can be used to tailor strength and ductility but is less common for typical mount applications compared to stress relief or HIP.
- standardy: Heat treatment processes for aerospace parts often need to comply with standards like AMS-H-81200 or specific customer requirements.
2. Support Structure Removal:
- Necessity: The support structures used during the build must be removed.
- Metody:
- Ruční odstranění: Supports designed with weak interfaces can sometimes be broken off manually or with simple hand tools. Requires careful handling to avoid damaging the part surface.
- Machining (Sawing, Milling, Grinding): Often required for robust supports or supports in tight locations. CNC machining provides precise control.
- Drátové elektroerozivní obrábění (EDM): Can be used to precisely cut supports close to the part surface, especially complex interfaces or internal supports, minimizing surface damage.
- Úvahy: DfAM plays a crucial role here – designing supports for easy access and removal significantly reduces post-processing time and cost. Areas where supports were attached often require further finishing.
3. Surface Finishing:
- Účel: To improve the as-built surface roughness, enhance fatigue life, meet aesthetic requirements, prepare for coatings, or achieve specific tolerances on mating surfaces.
- Common Methods (as detailed previously):
- Abrasive Blasting: Uniform matte finish, cleaning.
- Tumbling/Vibratory Finishing: Batch smoothing, edge breaking.
- CNC obrábění: For critical dimensions, GD&T features, sealing surfaces, and achieving smooth finishes (<1 µm Ra) on specific areas.
- Polishing (Manual/Automated): For very low Ra requirements.
- Electrochemical Polishing: For complex shapes.
- Impact on Fatigue: Surface roughness acts as initiation sites for fatigue cracks. Smoothing surfaces, particularly through methods that impart compressive residual stress (like shot peening, sometimes used after initial finishing), can significantly enhance fatigue performance – a critical factor for aerospace mounts under cyclic loading.
4. Hot Isostatic Pressing (HIP):
- What it is: A process where parts are subjected to high temperature (typically just below the melting point, e.g., ~900-950°C for Ti-6Al-4V) and high-pressure inert gas (e.g., Argon, typically 100-200 MPa) simultaneously in a specialized vessel.
- Why it’s Used (Especially for Aerospace):
- Eliminates Internal Porosity: The high pressure effectively closes internal voids (like gas porosity or lack-of-fusion defects) that may be present after printing, achieving near-full theoretical density (~100%).
- Improves Mechanical Properties: Significantly enhances ductility, fracture toughness, and most importantly, fatigue strength by removing potential crack initiation sites (pores).
- Reduces Property Scatter: Leads to more consistent and predictable material properties throughout the part and between different builds.
- Úleva od stresu: The high temperatures involved also effectively relieve residual stresses.
- Requirement: Due to the significant improvement in material integrity and fatigue performance, HIP is often a mandatory requirement for flight-critical 3D printed titanium components, including many aerospace mounts. Suppliers must have access to certified HIP capabilities.
5. Inspection and Testing (Quality Assurance):
- Necessity: To verify the part conforms to all specifications before it can be certified for flight.
- Nedestruktivní zkoušení (NDT):
- Visual Inspection (VT): Basic check for surface defects, correct form.
- Dimensional Metrology: CMM, 3D scanning (as detailed previously).
- Dye Penetrant Inspection (FPI/PT): Detects surface-breaking cracks or porosity.
- Radiographic Testing (RT – X-Ray) / Computed Tomography (CT): Detects internal defects (porosity, inclusions, cracks) and verifies internal geometries. CT is increasingly valuable for complex AM parts.
- Ultrazvukové testování (UT): Can detect subsurface defects.
- Destructive Testing (Typically on representative test coupons built alongside the parts):
- Tensile Testing: Measures yield strength, ultimate tensile strength, elongation (ductility).
- Fatigue Testing: Measures resistance to cyclic loading.
- Metallography: Microscopic examination of the material’s grain structure and density after processing.
- Chemical Analysis: Verifies alloy composition.
- Dokumentace: Rigorous documentation and traceability are required under aerospace quality systems like AS9100.
6. Optional Coatings:
- Depending on the specific application, mounts might receive additional coatings for:
- Zvýšená odolnost proti opotřebení: E.g., Tungsten Carbide or specialized hard coatings on contact surfaces.
- Thermal Barrier Coatings (TBCs): For parts operating in very high-temperature environments.
- Dry Film Lubricants: On mating surfaces to reduce friction.
- Priming/Painting: For environmental protection or identification.
Effectively managing this entire post-processing chain requires significant expertise, specialized equipment, and robust process control. When evaluating potential poskytovatelé služeb kovového 3D tisku, it’s essential to assess their in-house capabilities and their network of certified partners for steps like heat treatment, HIP, NDT, and precision machining.
Common Challenges in 3D Printing Titanium Mounts and How to Mitigate Them
While metal additive manufacturing offers substantial advantages for producing aerospace mounts, it’s not without its challenges. Understanding these potential issues and the strategies to mitigate them is crucial for successfully implementing AM technology for critical components. Proactive planning, process optimization, high-quality materials, and robust quality control are key to overcoming these hurdles. Engineers and procurement managers should be aware of these common metal AM challenges when specifying and sourcing 3D printed titanium parts.
1. Warping and Distortion:
- Výzva: Significant temperature gradients between the molten pool and surrounding material during printing induce internal stresses. As these stresses accumulate, they can cause the part to warp, distort, or even detach from the build plate, leading to build failure or parts outside dimensional tolerances. Titanium’s relatively low thermal conductivity can exacerbate this.
- Strategie zmírnění dopadů:
- Optimized Build Orientation: Orienting the part to minimize large flat surfaces parallel to the build plate and reduce Z-height can help.
- Robustní podpůrné struktury: Well-designed supports anchor the part firmly to the build plate and help conduct heat away.
- Simulace procesu: Using software to simulate the build process can predict areas of high stress and potential distortion, allowing for pre-compensation in the build file or adjustments to the support strategy.
- Optimalizované parametry procesu: Fine-tuning laser/beam power, scan speed, and hatching patterns can minimize thermal gradients.
- Build Plate Heating: Maintaining an elevated temperature in the build chamber (especially significant in EBM, but also used in some LPBF systems) reduces thermal gradients and lowers residual stress.
- Stress Relief Heat Treatment: Essential post-processing step to relieve built-up stresses before support removal.
2. Porosity:
- Výzva: The presence of small voids or pores within the printed material can significantly degrade mechanical properties, particularly fatigue strength, ductility, and fracture toughness. Porosity acts as stress concentrators where cracks can initiate. Types include:
- Lack-of-Fusion Porosity: Irregularly shaped voids caused by insufficient energy input to fully melt the powder particles or inadequate overlap between scan tracks.
- Keyhole Porosity / Gas Porosity: Spherical voids often caused by excessive energy input (vaporizing material that gets trapped) or gas trapped within the powder particles being released during melting.
- Strategie zmírnění dopadů:
- Optimalizované parametry procesu: Careful development and control of laser/beam power, scan speed, focus, layer thickness, and hatch spacing are critical to ensure complete melting and fusion without overheating.
- High-Quality Metal Powder: Using powder with high sphericity, controlled PSD, low internal gas content, and high purity is crucial. Poor quality powder (irregular shapes, satellites, internal porosity, contamination) is a major source of defects. Met3dp’s focus on producing premium kovové prášky using advanced Gas Atomization and PREP techniques directly addresses this challenge.
- Inert Atmosphere Control: Maintaining a high-purity inert gas atmosphere (Argon or Nitrogen) in the build chamber prevents oxidation and contamination during printing. Proper gas flow dynamics are needed to remove processing byproducts (spatter, fumes).
- Izostatické lisování za tepla (HIP): The most effective way to eliminate any remaining internal porosity after printing, leading to near-fully dense parts. Often mandatory for critical aerospace components.
3. Residual Stress:
- Výzva: Even if warping is controlled during the build, significant residual stresses remain locked within the as-built part. These stresses can lead to premature failure under load, reduced fatigue life, and unpredictable distortion during post-machining.
- Strategie zmírnění dopadů:
- Thermal Post-Processing: Stress relief heat treatment or HIP are the primary methods for significantly reducing residual stress.
- Optimalizace designu: Avoiding abrupt changes in cross-section and large solid volumes can help manage stress accumulation.
- Optimalizace parametrů procesu: As with porosity and warping, fine-tuned parameters can minimize stress build-up.
- Build Strategy: Scan strategies (e.g., island scanning, sector rotation) can distribute heat more evenly.
- Process Selection: EBM generally results in lower residual stress than LPBF due to the high, uniform temperature maintained throughout the build chamber.
4. Support Removal Difficulty & Surface Quality:
- Výzva: Removing support structures, especially intricate ones or those in internal cavities, can be time-consuming, costly, and potentially damage the part surface. The surfaces where supports were attached invariably have lower quality (witness marks, roughness).
- Strategie zmírnění dopadů:
- DfAM Focus: Design parts and select orientations to minimize the need for supports. Design supports with easily breakable or accessible interfaces. Design internal channels large enough for powder and support removal if needed.
- Specialized Removal Techniques: Utilizing appropriate tools (hand tools, CNC machining, wire EDM) based on the support type and location.
- Povrchová úprava: Plan for necessary finishing steps (blasting, tumbling, machining) to clean up support witness marks and achieve the required final surface quality.
5. Anisotropy (Directional Properties):
- Výzva: Due to the directional solidification of the melt pool and the resulting grain structure (often elongated columnar grains in the build direction), the mechanical properties (e.g., strength, ductility) of AM parts can vary depending on the direction of applied load relative to the build direction (X, Y vs. Z). This anisotropy needs to be accounted for in design and analysis.
- Strategie zmírnění dopadů:
- Characterization & Understanding: Testing material properties in different orientations relative to the build direction to understand the degree of anisotropy for the specific process and material.
- Optimized Build Orientation: Aligning the primary load direction during service with the orientation exhibiting the most favorable properties (often perpendicular to the build direction/parallel to layers).
- Post-Processing (HIP): HIP can help to partially homogenize the microstructure, reducing the degree of anisotropy, although it may not eliminate it entirely.
- Process Selection: Different AM processes can result in varying degrees of anisotropy.
6. Powder Handling and Safety:
- Výzva: Fine metal powders, especially reactive materials like titanium, pose safety risks. They can be flammable or explosive under certain conditions (dust clouds) and pose inhalation risks. Contamination (e.g., by oxygen or moisture) can degrade powder quality.
- Strategie zmírnění dopadů:
- Inert Atmosphere Handling: Handling titanium powder under an inert gas (Argon) environment whenever possible.
- Proper Grounding: Preventing static electricity buildup.
- Osobní ochranné prostředky (OOP): Respirators, gloves, eye protection.
- Good Housekeeping: Preventing powder accumulation.
- Controlled Environment: Maintaining low humidity storage conditions.
- Školení: Ensuring personnel are trained in safe powder handling procedures.
7. Cost and Scalability:
- Výzva: Metal AM can have high capital equipment costs and relatively slower build rates compared to some traditional mass production methods. Powder costs, especially for high-quality aerospace grades, can also be significant. This can make AM less cost-effective for very simple parts or extremely high production volumes where traditional methods are well-established.
- Strategie zmírnění dopadů:
- Focus on High-Value Applications: Target components where AM’s benefits (complexity, weight reduction, consolidation, reduced lead time for complex parts) provide the most significant value proposition, justifying the cost. Aerospace mounts often fall into this category.
- DfAM for Cost Reduction: Optimize designs to minimize material usage and build time (e.g., topology optimization, support minimization).
- Nesting: Printing multiple parts simultaneously on the build plate to maximize machine utilization.
- Process Efficiency: Utilizing high-productivity machines (e.g., multi-laser systems) and optimizing process parameters.
- Supplier Collaboration: Working with experienced AM service providers who have optimized workflows and economies of scale.
By acknowledging these challenges and implementing appropriate mitigation strategies throughout the design, manufacturing, and post-processing stages, companies can successfully leverage metal 3D printing to produce high-quality, reliable titanium aerospace mounts that meet the rigorous demands of the industry.
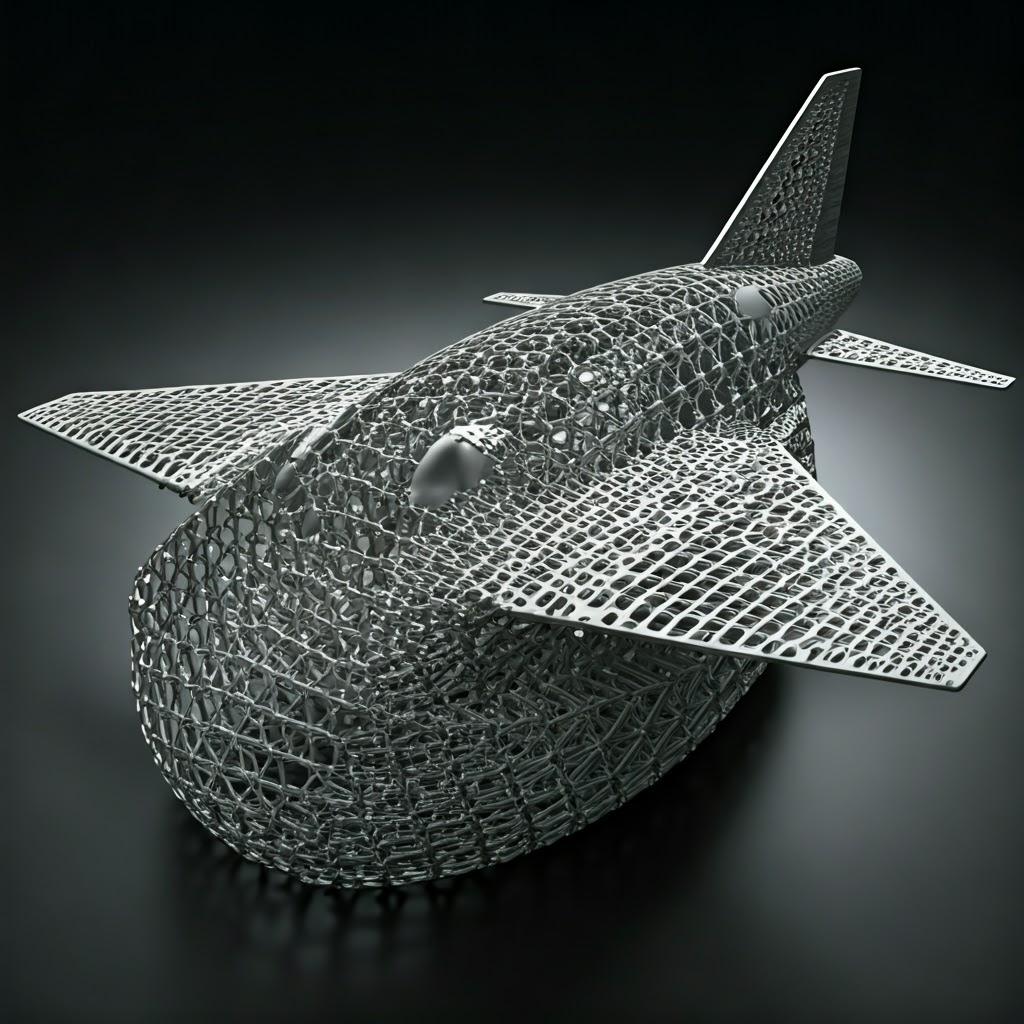
How to Choose the Right Metal 3D Printing Service Provider for Aerospace Mounts
Selecting the right manufacturing partner is arguably as critical as the design and material selection when producing 3D printed titanium mounts for aerospace applications. The unique demands of the aerospace industry – stringent quality requirements, complex components, safety-critical functions, and rigorous certification processes – mean that not all metal additive manufacturing service providers are created equal. Choosing an unqualified supplier can lead to costly delays, non-conforming parts, and potential risks. Procurement managers and engineers must conduct thorough due diligence to identify a partner with the specific expertise, certifications, capabilities, and quality mindset required for aerospace. Here’s a guide to evaluating potential suppliers:
1. Proven Aerospace Expertise and Experience:
- Track Record: Look for providers with demonstrable experience in manufacturing components, specifically structural parts like mounts, for the aerospace industry. Ask for case studies, references, and examples of similar parts they have produced.
- Material Specialization: Deep expertise in processing Ti-6Al-4V and Ti-6Al-4V ELI is essential. This includes understanding its metallurgy, appropriate AM process parameters, heat treatment responses, and common failure modes.
- Application Understanding: The supplier should understand the functional requirements of aerospace mounts (load conditions, fatigue life, environmental factors) and how AM can best meet these needs.
- Regulatory Awareness: Familiarity with relevant aerospace standards (AMS, ASTM, AS), quality clauses (e.g., from Boeing, Airbus, Lockheed Martin, NASA), and potentially ITAR (International Traffic in Arms Regulations) or other export control requirements if applicable.
2. Essential Certifications:
Certifications are non-negotiable proof points of a supplier’s commitment to quality and process control, especially in aerospace.
- AS9100: This is the cornerstone Quality Management System (QMS) standard for the Aviation, Space, and Defense industries. It incorporates ISO 9001 requirements but adds numerous aerospace-specific controls related to safety, reliability, traceability, risk management, configuration management, and more. An AS9100 certified 3D printing supplier is typically considered a minimum requirement for flight-critical components.
- ISO 9001: A foundational QMS certification, indicating established processes for quality control, documentation, and continuous improvement. Often a prerequisite for AS9100.
- Nadcap Accreditation: While AS9100 covers the overall QMS, Nadcap provides specific accreditation for special processes. Look for Nadcap accreditation (either in-house or through certified partners) for critical post-processing steps like:
- Heat Treating (HT)
- Non-Destructive Testing (NDT)
- Welding (WLD) – Less common for AM parts themselves but may apply to associated processes.
- Materials Testing Laboratories (MTL)
- Chemical Processing (CP) – For certain surface treatments or etching. (Note: Nadcap for Additive Manufacturing itself is evolving but accreditations for the associated special processes are crucial).
3. Technology, Equipment, and Capacity:
- Vhodná technologie AM: Ensure the supplier has the right type of AM technology for your needs (e.g., LPBF for fine features, EBM for potentially lower stress and different microstructures). Assess the specific make/model of printers, their condition, and maintenance records. Companies like Met3dp, offering their own advanced tiskárny SEBM alongside LPBF capabilities, provide technological options. You can learn more O nás and our commitment to industry-leading equipment.
- Machine Calibration & Maintenance: Rigorous protocols for machine calibration, preventative maintenance, and performance monitoring are vital for consistent part quality and accuracy.
- Capacity and Redundancy: Does the supplier have sufficient machine capacity to meet your project timelines, including potential ramp-up for production volumes? Do they have multiple machines to provide redundancy in case of downtime?
- Controlled Environment: Proper environmental controls (temperature, humidity, cleanliness) in the production facility are important, especially for handling sensitive metal powders.
4. Material Expertise and Quality Control:
- Powder Sourcing and Management: How does the supplier source, test, and handle titanium powder?
- Sourcing: Do they buy from qualified external suppliers, or do they have in-house production like Met3dp, offering greater control over quality and traceability?
- Incoming Inspection: What tests are performed on incoming powder batches (chemistry, PSD, morphology, flowability)?
- Traceability: Can they trace a specific part back to the exact powder batch used?
- Handling & Storage: Are strict procedures followed for inert atmosphere handling, storage, and preventing cross-contamination?
- Recycling Strategy: What are their validated procedures for sieving, testing, and blending reused powder to ensure continued quality? Strict control over powder lifecycle management is critical for aerospace.
5. Comprehensive In-House Capabilities & Partner Network:
Producing a finished aerospace mount requires more than just printing. Evaluate the supplier’s ability to manage the entire workflow:
- DfAM Support: Do they offer engineering support to help optimize designs for additive manufacturing?
- Tisk: Core capability with robust process control.
- Následné zpracování: Assess their in-house capabilities for stress relief/heat treatment, support removal, surface finishing, and crucial steps like HIP. If outsourced, are their partners Nadcap certified and tightly integrated into their quality system?
- Obrábění: Do they have in-house precision CNC machining capabilities or qualified partners to finish critical features?
- Inspection & Metrology: Do they possess advanced metrology equipment (CMM, 3D scanners, CT scanners) and certified NDT capabilities (FPI, X-ray)?
An integrated service offering often leads to better communication, shorter lead times, and clearer accountability.
6. Engineering Support and Collaboration:
- Application Engineers: Access to knowledgeable engineers who can provide DfAM consultation, advise on material selection, simulate build processes, and support qualification efforts is invaluable.
- Collaborative Approach: Look for a partner willing to work closely with your team, providing transparency and clear communication throughout the project lifecycle.
7. Robust Quality Management System (QMS):
- Beyond the Certificate: Look for evidence of a deeply ingrained quality culture. This includes robust process documentation, operator training records, clear non-conformance reporting and corrective action procedures, rigorous configuration management, and detailed data collection/retention.
8. Lead Times, Capacity, and Responsiveness:
- Realistic Quoting: Do they provide clear and realistic lead time estimates based on current capacity?
- Meeting Deadlines: What is their track record for on-time delivery?
- Škálovatelnost: Can they support your needs from prototyping through potential series production?
Evaluation Checklist Summary:
Kritéria | Klíčové úvahy | Důležitost |
---|---|---|
Aerospace Experience | Proven track record, Ti64 expertise, application understanding, regulatory awareness. | Kritické |
Certifikace | AS9100 (mandatory), ISO 9001, Nadcap (for special processes). | Kritické |
Technologie a vybavení | Appropriate AM tech (LPBF/EBM), machine quality & maintenance, capacity, redundancy. | Vysoký |
Material Control | Powder sourcing, testing, traceability, handling, recycling protocols. (In-house production like Met3dp is a plus). | Kritické |
End-to-End Capability | DfAM, Printing, Heat Treat, HIP, Machining, Finishing, NDT, Metrology (in-house or certified partners). | Kritické |
Technická podpora | DfAM consultation, process simulation, qualification support. | Vysoký |
Quality Management System | Documented processes, traceability, risk management, continuous improvement culture beyond the certificate. | Kritické |
Lead Time & Capacity | Realistic quotes, on-time delivery track record, scalability. | Vysoký |
Communication/Partnership | Transparency, responsiveness, collaborative approach. | Vysoký |
Export do archů
By carefully evaluating potential suppliers against these criteria, aerospace companies can confidently select a capable and reliable aerospace component manufacturing partner equipped to deliver high-quality, flight-worthy 3D printed titanium mounts.
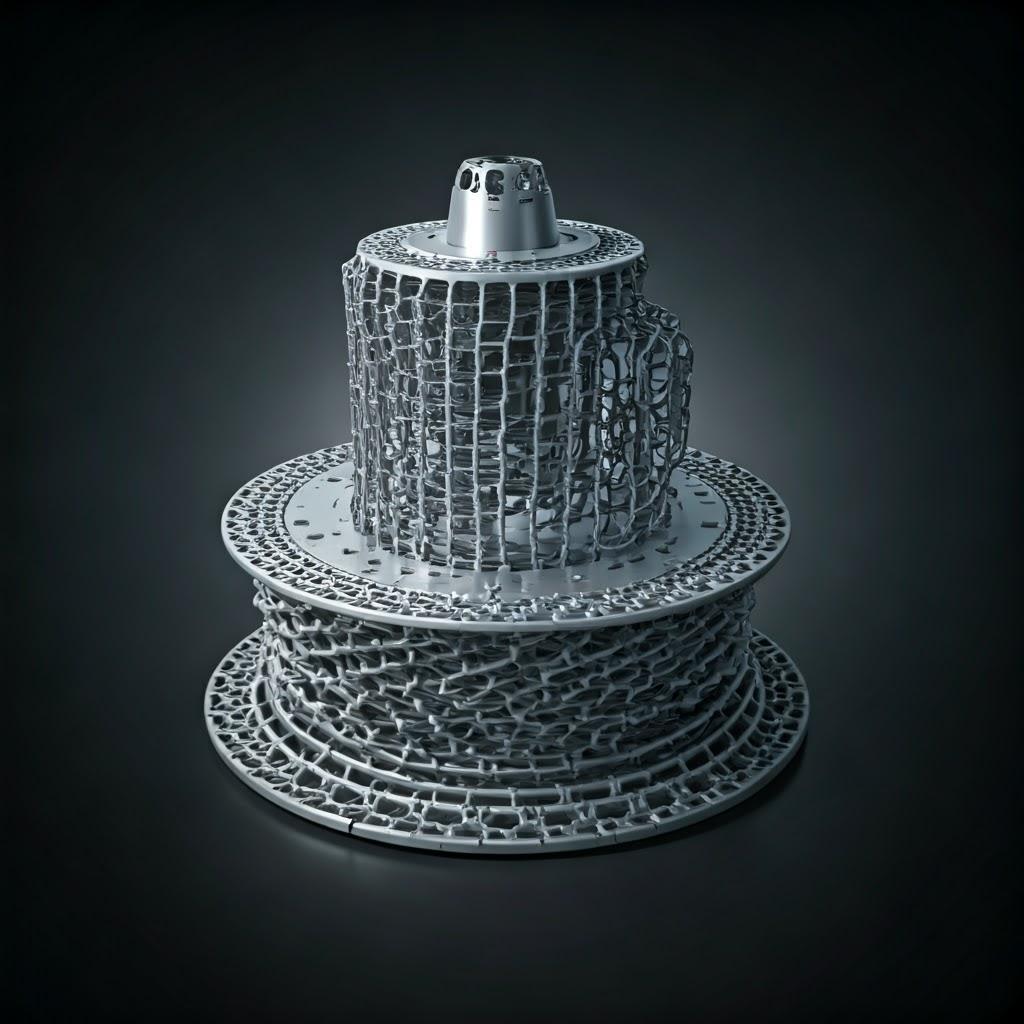
Cost Factors and Lead Time for 3D Printed Titanium Aerospace Mounts
While the performance and design benefits of 3D printed titanium mounts are clear, understanding the associated costs and typical lead times is essential for project planning, budgeting, and comparing AM to traditional manufacturing methods. Both cost and lead time are influenced by a complex interplay of factors related to the material, design complexity, machine time, labor, post-processing, and quality requirements inherent in aerospace. Procurement managers need a clear picture of these drivers to make informed sourcing decisions and manage expectations.
Key Cost Drivers for 3D Printed Titanium Mounts:
The final price of a 3D printed titanium mount is derived from several contributing factors:
Cost Driver | Description & Influencing Factors | Impact on Overall Cost |
---|---|---|
1. Material Cost | Price per kilogram of high-quality, aerospace-grade Ti-6Al-4V or Ti-6Al-4V ELI powder. Includes the volume of the part itself plus the volume of support structures. Powder refresh rates and recycling efficiency also play a role. Titanium powder is inherently expensive. | Vysoký |
2. Machine Time | Cost per hour of operating the metal AM machine multiplied by the total build time. Build time is primarily driven by: <br> – Part Volume: Larger parts take longer. <br> – Part Height (Z-axis): More layers mean longer print times. <br> – Complexity: Intricate scan paths can increase time per layer. <br> – Nesting Efficiency: How many parts fit onto a single build plate. | Vysoký |
3. Labor Costs | Skilled labor required for: <br> – Build Preparation: File setup, slicing, support generation, machine setup. <br> – Machine Operation & Monitoring. <br> – Part Removal/Depowdering: Extracting parts from the build chamber, removing bulk powder. <br> – Support Removal: Often manual or semi-automated, can be very time-consuming for complex supports. <br> – Post-Processing Labor: Machining, finishing, inspection tasks. | Významné |
4. Post-Processing Costs | Costs associated with necessary steps after printing: <br> – Stress Relief / Heat Treatment: Furnace time, energy, process control. <br> – Hot Isostatic Pressing (HIP): Often outsourced to specialized facilities, a significant cost addition but critical for properties. <br> – Machining: CNC machine time and labor for critical tolerances/finishes. <br> – Surface Finishing: Blasting, tumbling, polishing, etc. <br> – NDT & Inspection: Equipment time, certified inspector labor. | Významné |
5. Design Complexity & Supports | While geometric complexity itself doesn’t drastically increase print cost (unlike machining), highly complex designs may necessitate extensive support structures. This increases: <br> – Material Consumption (supports). <br> – Build Time (supports add volume/height). <br> – Post-Processing Labor (support removal). | Střední až vysoká |
6. Quality Assurance & Certification | Costs associated with the rigorous documentation, traceability, testing (destructive & NDT), inspection reports, and certification paperwork required to meet aerospace standards (e.g., AS9100 compliance, First Article Inspection Reports – FAIR). | Střední |
7. Order Volume | AM cost scaling differs from traditional methods. <br> – Setup costs (file prep, build planning) are amortized over the batch size. <br> – Full build plates are more machine-time efficient. <br> – Potential for volume discounts from suppliers on wholesale 3D printing orders. <br> – However, per-part cost reduction with volume is generally less dramatic than with high-volume methods like casting or stamping. | Střední |
8. Design Iterations | Costs associated with prototyping and design changes during the development phase. AM excels at rapid iteration, potentially reducing overall development cost compared to methods requiring tooling. | Variable (Development) |
Export do archů
Understanding the Cost Calculation: AM service providers typically calculate costs based on a combination of material consumption (by weight or volume), machine time (based on build simulation), and estimated labor/post-processing efforts. Providing a clean, optimized CAD model and clear specifications helps ensure accurate quoting.
Lead Time Components and Typical Ranges:
Lead time – the total time from order placement to part delivery – is often as critical as cost in aerospace, especially for MRO or rapid development programs. It comprises several stages:
- Quoting & Order Processing (1-5 Business Days): Reviewing the CAD model and specifications, generating a quote, confirming the order, and performing initial build preparation (e.g., orientation, support strategy planning).
- Queue Time (Variable: Days to Weeks): Time spent waiting for an appropriate machine to become available. This depends heavily on the supplier’s current workload and capacity.
- Print Time (Hours to Several Days): The actual time the part(s) spend printing in the AM machine. Dependent on part size, height, complexity, and nesting density. A single large mount or a full plate of smaller mounts can easily take 24-72 hours or more to print.
- Cool Down (Several Hours): Time required for the build chamber and parts to cool sufficiently before removal, often done under an inert atmosphere.
- Post-Processing (Variable: Days to Weeks): This is often the most significant and variable component of the lead time. It includes:
- Stress Relief / Heat Treatment (can take 1-3 days including furnace cycles and handling).
- HIP (requires scheduling with HIP providers, transport time, and the HIP cycle itself – can add 1-2 weeks).
- Support Removal & Basic Finishing (1-3 days depending on complexity).
- Precision Machining (depends heavily on complexity, can add several days to weeks).
- NDT Inspection & Quality Checks (1-5 days depending on requirements).
- Shipping (Variable): Transit time to the customer’s location.
Typical Lead Time Ranges (Indicative):
- Prototypes (with basic post-processing like stress relief): 2 – 4 týdny
- Functional Prototypes / Qualification Parts (including HIP, basic machining): 4 – 7 weeks
- Production Parts (fully processed, inspected, certified): 5 – 10+ weeks
Important Note (As of April 16, 2025): Lead times can fluctuate based on overall industry demand, specific supplier backlogs, and availability of specialized post-processing services like HIP. Always confirm current lead times with your chosen supplier during the quoting stage.
Strategies for Optimizing Cost and Lead Time:
- Design for AM (DfAM): Optimize designs to minimize volume, reduce build height, and minimize support structures. Consolidate parts to reduce downstream assembly and inspection.
- Nesting: Maximize the number of parts printed per build plate.
- Minimize Post-Processing: Design parts to minimize the need for extensive machining or complex support removal. Clearly define which features require tight tolerances or specific finishes.
- Clear Specifications: Provide complete and unambiguous drawings and specifications upfront to avoid delays during quoting or production.
- Supplier Collaboration: Engage with your AM provider early in the design process to leverage their expertise for cost and lead time optimization.
By understanding these cost and lead time drivers, aerospace companies can better plan projects, manage budgets, and leverage the full potential of 3D printed titanium mounts effectively.
Frequently Asked Questions (FAQ) about 3D Printed Titanium Aerospace Mounts
Here are answers to some common questions engineers and procurement managers have when considering additive manufacturing for titanium aerospace mounts:
Q1: Are 3D printed titanium mounts as strong as traditionally machined or forged ones?
A: Yes, when produced using qualified processes and appropriate post-processing, 3D printed Ti-6Al-4V components can exhibit mechanical properties (like tensile strength, yield strength, and fatigue strength) that are comparable to, and sometimes even exceed, those of wrought (machined from billet) or forged counterparts. Achieving this requires:
- Optimized AM process parameters to ensure full density and good layer bonding.
- High-quality, aerospace-grade powder with controlled chemistry and morphology.
- Essential post-processing, particularly Izostatické lisování za tepla (HIP), which eliminates internal porosity and significantly enhances fatigue properties, often making them equivalent or better than wrought materials, especially regarding fatigue life consistency.
- Appropriate stress relief or annealing heat treatments. Performance depends heavily on the quality control implemented by the aerospace additive manufacturing supplier.
Q2: What specific aerospace certifications are absolutely necessary for suppliers producing 3D printed mounts?
A: The primary certification for the supplier’s Quality Management System is AS9100. This demonstrates they have the necessary controls for traceability, configuration management, risk management, and other processes critical for aerospace. Beyond AS9100, Nadcap accreditation is crucial for any special processes involved, whether performed in-house or by subcontractors. This commonly includes Heat Treating (HT) a Non-Destructive Testing (NDT). If HIP is used (which is highly recommended for critical mounts), the HIP provider should also hold relevant certifications (often ISO 9001/AS9100 and potentially Nadcap HT). Part qualification itself involves rigorous testing and documentation specific to the component and customer requirements, often referencing AMS or specific OEM standards.
Q3: Can we simply take our existing mount design (made for machining) and 3D print it?
A: While it might be technically possible to print a design originally intended for machining, doing so misses out on the primary advantages of additive manufacturing. A direct “print-as-is” approach often results in a part that is heavier and potentially more expensive to produce via AM than necessary. To truly benefit from AM, the part should be redesigned using Design for Additive Manufacturing (DfAM) principles. This involves:
- Running topology optimization to remove unnecessary material based on load paths.
- Considering part consolidation opportunities to reduce assembly complexity.
- Optimizing the design to minimize support structures and facilitate post-processing.
- Leveraging AM’s ability to create complex internal features or lattice structures for weight reduction or enhanced functionality. Simply printing the old design is rarely the optimal approach.
Q4: What is the typical cost saving when using AM for aerospace mounts compared to machining?
A: Cost savings are highly variable and depend heavily on the specific component, its complexity, the traditional manufacturing buy-to-fly ratio, and production volume.
- Direct Part Cost: For simple mounts produced in high volumes, traditional machining might still be cheaper on a direct part-vs-part basis. However, for highly complex mounts, AM can be competitive or even cheaper due to design freedom and reduced setup costs.
- Material Waste Reduction: AM significantly reduces material waste compared to machining titanium billets (poor buy-to-fly ratio). Savings on expensive titanium raw material can be substantial (50-90% reduction in waste).
- Snížení hmotnosti: AM enables significant weight savings (20-60%) through topology optimization. This translates to substantial operational cost savings over the aircraft’s lifetime (fuel burn) or increased revenue potential (payload). This lifecycle saving often outweighs any potential increase in initial part cost.
- Konsolidace částí: Reducing part count saves on assembly labor, fasteners, inventory management, and potential failure points.
- Zkrácení dodací lhůty: Faster prototyping and potential for on-demand spares offer indirect cost savings. The true value proposition often lies in the combination of reduced material waste, lifecycle operational savings from weight reduction, and design/performance improvements, rather than solely on lower initial manufacturing cost in all cases.
Q5: How does Met3dp ensure the quality of its titanium powders (like Ti-6Al-4V) specifically for demanding aerospace applications?
A: Met3dp recognizes that powder quality is the foundation for reliable aerospace additive manufacturing. We ensure excellence through multiple strategies:
- Advanced Production Technologies: Utilizing industry-leading Gas Atomization (VIGA) and Plasma Rotating Electrode Process (PREP) technologies designed to produce highly spherical powders with minimal satellites and high purity.
- Strict Raw Material Control: Sourcing high-quality input materials and controlling chemistry precisely throughout the melting process.
- In-Process Controls: Monitoring key parameters during atomization to ensure consistency.
- Rigorous Quality Control Testing: Each powder batch undergoes extensive testing for:
- Chemical Composition (including critical interstitial elements like Oxygen/Nitrogen).
- Particle Size Distribution (PSD) using laser diffraction.
- Morphology and Sphericity (analyzed via SEM imaging).
- Flowability (e.g., Hall Flowmeter testing).
- Apparent and Tap Density.
- Batch Traceability: Maintaining complete traceability from raw materials to the final packaged powder.
- Optimized Packaging: powders are packaged under inert conditions to prevent contamination and ensure long shelf life. This comprehensive approach ensures that Met3dp’s Ti-6Al-4V and other kovové prášky consistently meet the stringent requirements for density, purity, flowability, and morphology demanded by critical aerospace applications, enabling our customers to print high-quality, reliable components.
Conclusion: Elevating Aerospace Structures with 3D Printed Titanium Mounts
The landscape of aerospace manufacturing is undergoing a profound transformation, driven by the relentless pursuit of lighter, stronger, and more efficient components. In this evolution, metal additive manufacturing combined with high-performance titanium alloys like Ti-6Al-4V and Ti-6Al-4V ELI has emerged as a pivotal technology, particularly for critical components like aerospace mounts. As we’ve explored, the ability to 3D print these vital structural elements offers compelling advantages that directly address the core challenges of the aerospace industry.
We’ve seen how AM unshackles designers from the constraints of traditional manufacturing, enabling the creation of topologically optimized, lightweight mounts that were previously inconceivable. This design freedom translates directly into significant úspora hmotnosti, improving fuel efficiency and payload capacity – key metrics for aircraft and spacecraft performance. The capability for konsolidace částí, replacing complex assemblies with single, monolithic printed components, further reduces weight while simultaneously simplifying assembly, reducing part count, and eliminating potential failure points associated with joints and fasteners.
Beyond weight and complexity, metal AM delivers účinnost materiálu far superior to subtractive machining, drastically reducing the waste of expensive titanium and improving the crucial buy-to-fly ratio. The technology also accelerates innovation cycles through rychlé prototypování and offers the potential for výroba na vyžádání of spare parts, enhancing supply chain resilience and reducing MRO lead times. When combined with essential post-processing steps like Izostatické lisování za tepla (HIP), 3D printed titanium mounts demonstrate mechanical properties comparable or superior to their conventionally manufactured counterparts, ensuring the structural integrity and reliability demanded by flight-critical applications.
However, successfully leveraging this technology requires careful consideration of DfAM principles, a thorough understanding of process capabilities and limitations regarding tolerances and surface finish, diligent management of post-processing requirements, and proactive mitigation of potential challenges like residual stress and porosity.
Crucially, the success of implementing 3D printed titanium aerospace mounts hinges on selecting the right partners. This means choosing material suppliers who guarantee the highest standards of powder quality and consistency, and partnering with additive manufacturing service providers who possess proven aerospace expertise, essential certifications (like AS9100 and relevant Nadcap accreditations), robust quality management systems, and comprehensive end-to-end capabilities from design support through printing, post-processing, and rigorous inspection.
Met3dp stands at the forefront of this technological advancement, offering a unique combination of capabilities as both a developer of advanced Selective Electron Beam Melting (SEBM) printers and a manufacturer of high-quality, spherical metal powders produced via cutting-edge Gas Atomization and PREP technologies. Our deep expertise in materials science and additive manufacturing processes, coupled with our commitment to quality and reliability, makes us an ideal partner for aerospace companies seeking to implement 3D printing and accelerate their digital manufacturing transformations.
The adoption of 3D printed titanium mounts is more than just a new manufacturing technique; it’s a strategic enabler for next-generation aerospace design and performance. It allows engineers to build lighter, fly farther, carry more, and innovate faster.
Ready to explore how additive manufacturing can elevate your aerospace structures? Kontakt Met3dp today to discuss your specific component needs and discover how our comprehensive solutions – from industry-leading printers and premium powders to expert application development services – can power your organization’s additive manufacturing goals and help you reach new heights.
Sdílet na
MET3DP Technology Co., LTD je předním poskytovatelem řešení aditivní výroby se sídlem v Qingdao v Číně. Naše společnost se specializuje na zařízení pro 3D tisk a vysoce výkonné kovové prášky pro průmyslové aplikace.
Dotaz k získání nejlepší ceny a přizpůsobeného řešení pro vaše podnikání!
Související články
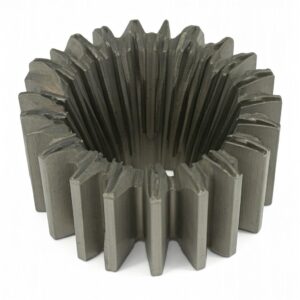
Vysoce výkonné segmenty lopatek trysek: Revoluce v účinnosti turbín díky 3D tisku z kovu
Přečtěte si více "O Met3DP
Nedávná aktualizace
Náš produkt
KONTAKTUJTE NÁS
Nějaké otázky? Pošlete nám zprávu hned teď! Po obdržení vaší zprávy obsloužíme vaši žádost s celým týmem.
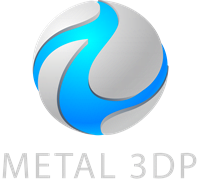
Kovové prášky pro 3D tisk a aditivní výrobu
SPOLEČNOST
PRODUKT
kontaktní informace
- Město Qingdao, Shandong, Čína
- [email protected]
- [email protected]
- +86 19116340731