High-Temp Jet Engine Liners via 3D Printing
Obsah
Introduction: Revolutionizing Jet Engine Liners with Metal Additive Manufacturing
The aerospace industry is relentlessly driven by the pursuit of higher efficiency, reduced emissions, and enhanced performance. At the heart of this pursuit lies the gas turbine engine, a marvel of engineering operating under extreme conditions. Within these engines, components like the combustion liner face some of the most demanding environments imaginable – scorching temperatures, high pressures, and corrosive gases. Traditionally, manufacturing these intricate, high-performance parts involved complex, multi-step processes like casting, forging, and extensive machining, often leading to long lead times, significant material waste, and limitations in design complexity.
Enter metal additive manufacturing (AM), more commonly known as 3D tisk z kovu. This transformative technology is rewriting the rules for designing and producing critical aerospace components, including jet engine liners. By building parts layer by layer directly from metal powder based on a digital model, AM unlocks unprecedented design freedom, enables rapid prototyping and iteration, reduces material waste, and consolidates multiple parts into single, optimized components. For high-temperature applications like combustion liners, the ability to utilize advanced superalloys and create complex internal cooling channels offers a pathway to lighter, more durable, and more efficient engines. Companies at the forefront of this revolution, like Met3dp, leverage decades of expertise in both advanced metal powders and cutting-edge printing systems to deliver components that meet the stringent demands of the aerospace sector. This shift towards additive manufacturing isn’t just an alternative; it’s a fundamental enabler for next-generation gas turbine technology, promising enhanced performance and a more sustainable future for aviation. As engineers and procurement managers seek reliable suppliers and wholesale solutions for these advanced components, understanding the capabilities and benefits of metal AM becomes crucial.
Critical Functions: The Role of Liners in Modern Jet Engines
The combustion liner, often referred to simply as the “liner” or “combustor liner,” is a critical component situated within the combustor section of a gas turbine engine. Its primary function is to contain the high-temperature combustion process, protecting the surrounding engine components (like the turbine casing and structural elements) from the extreme heat generated – often exceeding 1500∘C (2732∘F). But its role extends far beyond simple containment.
Key Functions of a Jet Engine Liner:
- Containment and Thermal Shielding: The liner forms the boundary for the combustion zone, ensuring the intense flame is kept away from thermally sensitive parts of the engine. It must withstand direct flame impingement and extreme thermal gradients.
- Mixing Zone Definition: It plays a crucial role in guiding the airflow within the combustor. It helps to properly mix the incoming compressed air with fuel for efficient combustion and directs the flow of cooling air. Precisely controlled mixing is essential for achieving stable combustion, minimizing pollutant formation (like NOx), and ensuring uniform temperature distribution at the turbine inlet.
- Structural Integrity: Despite the extreme temperatures, the liner must maintain its structural shape and integrity under significant pressure differentials and aerodynamic loads throughout the engine’s operational envelope, from takeoff thrust to cruise conditions. Buckling, cracking, or distortion can lead to catastrophic engine failure.
- Durability and Longevity: Liners are subjected to severe thermal cycling during engine start-up, operation, and shutdown. This repeated expansion and contraction induces thermal fatigue. They must also resist oxidation and corrosion from the combustion gases and potential fuel impurities over thousands of flight hours. Reliability is paramount, making material selection and manufacturing quality critical concerns for aerospace parts manufacturers and suppliers.
- Cooling Management: Modern liners incorporate sophisticated cooling schemes (e.g., effusion cooling, film cooling) using thousands of precisely placed small holes or intricate internal passages. These schemes use a portion of the compressor discharge air to form protective layers of cooler air along the liner walls, drastically reducing the metal temperature and extending component life. The effectiveness of this cooling directly impacts engine efficiency and liner durability.
The performance of the combustion liner directly influences several key engine parameters:
- Engine Efficiency: Proper mixing and controlled combustion within the liner contribute to higher thermal efficiency. Effective cooling allows for higher turbine inlet temperatures, a key driver for improving the engine’s overall thermodynamic efficiency and reducing fuel consumption.
- Emise: The design of the liner significantly impacts the formation of pollutants like Nitrogen Oxides (NOx), Carbon Monoxide (CO), and unburned hydrocarbons (UHC). Optimized fuel-air mixing patterns can drastically reduce harmful emissions.
- Reliability and Maintenance Costs: A durable, well-designed liner extends the time between engine overhauls, reducing maintenance costs and increasing aircraft availability. Sourcing high-quality liners from reliable gas turbine component suppliers is essential for airlines and MRO (Maintenance, Repair, Overhaul) providers.
Given these critical functions and the demanding operating environment, manufacturing jet engine liners requires advanced materials and precision manufacturing techniques capable of producing complex geometries with high fidelity and material integrity. This is where the unique advantages of metal additive manufacturing truly shine.
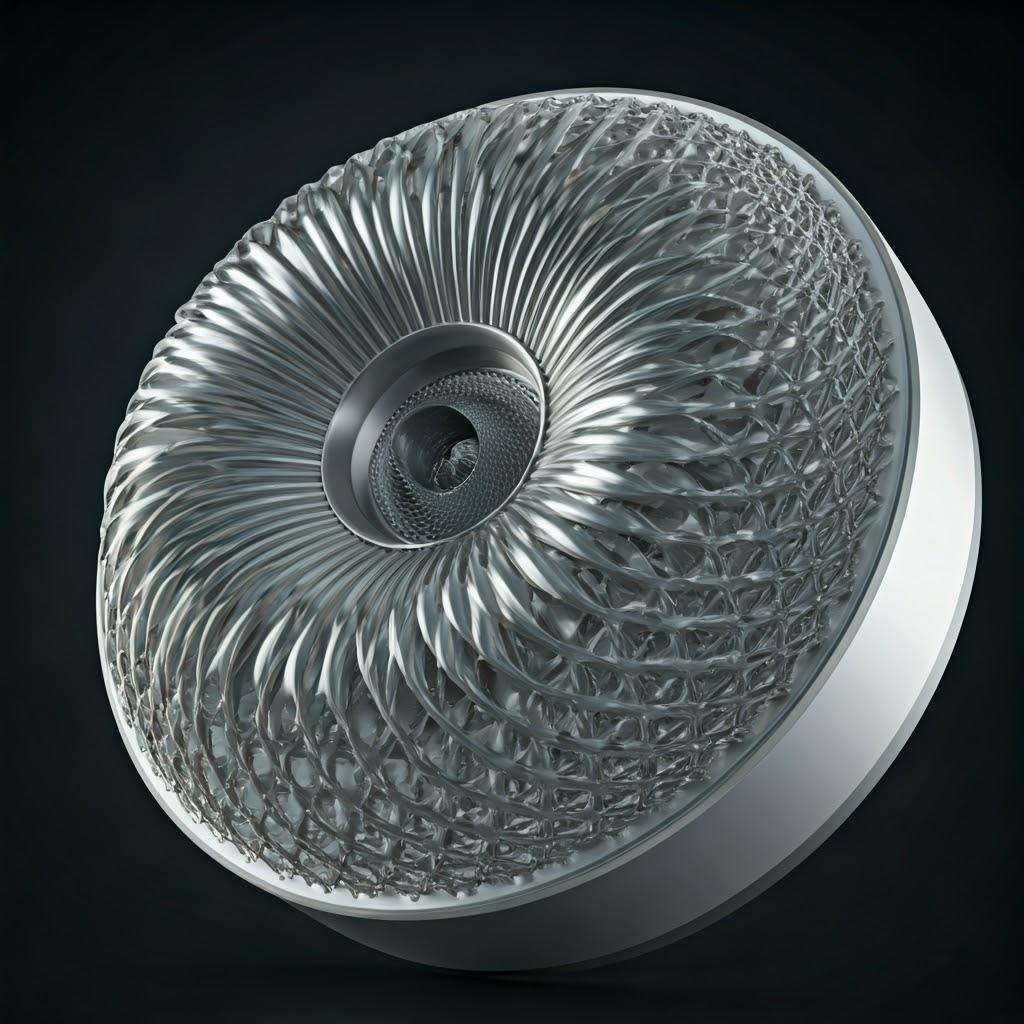
Why Metal 3D Printing for Jet Engine Liners? Unlocking Performance Gains
Traditional manufacturing methods for jet engine liners, primarily involving sheet metal fabrication (forming, welding, brazing) or investment casting, have served the industry for decades. However, they inherently possess limitations that metal additive manufacturing can overcome, offering significant advantages for these high-value components. The complex shapes, intricate cooling features, and demanding material requirements of modern liners make them ideal candidates for AM processes like Selektivní laserové tavení (SLM) or Electron Beam Melting (EBM).
Key Advantages of Using Metal AM for Liners:
- Unprecedented Design Freedom & Complexity:
- Intricate Cooling Geometries: AM allows the creation of highly complex internal cooling channels, optimized effusion cooling patterns (precisely shaped and oriented holes), and lattice structures that are impossible or prohibitively expensive to produce using traditional methods. This enables more effective cooling, allowing for higher combustion temperatures and thus improved engine efficiency and thrust-to-weight ratio.
- Optimized Shapes: Techniques like topology optimization can be employed to remove material from low-stress areas, resulting in lighter liners without compromising structural integrity. Generative design algorithms can explore novel shapes optimized for flow mixing and thermal management.
- Konsolidace částí:
- Reduced Assembly Complexity: Liners traditionally fabricated from multiple sheet metal pieces require extensive welding or brazing, introducing potential failure points and increasing manufacturing complexity. AM allows for the consolidation of numerous parts into a single, monolithic component.
- Improved Structural Integrity: Eliminating joints and welds enhances the overall strength and reliability of the liner, reducing the risk of cracks initiating at weld seams under thermal cycling.
- Lower Part Count & Logistics: Consolidating parts simplifies inventory management, assembly processes, and supply chain logistics for aerospace manufacturers.
- Accelerated Development & Prototyping:
- Rapid Iteration: AM enables designers to quickly produce and test multiple design variations of a liner. This rapid prototyping capability significantly shortens the development cycle for new engine programs or upgrades, allowing for faster innovation and performance improvements.
- Reduced Tooling Costs: Unlike casting or forming, AM does not require expensive molds or dies, making it cost-effective for low-volume production runs typical during development and testing phases, and even for producing customized or legacy parts.
- Material Efficiency & Waste Reduction:
- Near-Net Shape Manufacturing: AM builds parts layer by layer, using only the material necessary for the component and required support structures. This contrasts sharply with subtractive manufacturing (machining from billet), which can generate substantial material waste, particularly with expensive superalloys.
- Udržitelnost: Reduced material consumption aligns with industry goals for more sustainable manufacturing practices.
- Enhanced Performance Potential:
- Odlehčení: Through topology optimization and the use of complex geometries like internal lattices, AM can significantly reduce the weight of the liner, contributing to overall engine weight reduction – a critical factor for improving fuel efficiency and aircraft performance.
- Improved Durability: Optimized cooling and monolithic designs can lead to lower operating metal temperatures and reduced stress concentrations, potentially extending the operational life of the liner and improving engine reliability. B2B buyers focused on total cost of ownership benefit from this increased durability.
- Advanced Material Utilization:
- Superalloy Processing: AM techniques like SLM and EBM are well-suited for processing high-performance nickel-based superalloys (like IN738LC and Hastelloy X) required for the extreme temperatures in the combustor. Companies like Met3dp specialize in producing atomized powders of these alloys optimized for AM processes, ensuring high density and excellent material properties in the final part. Learn more about the different tiskových metod suitable for these materials.
Comparison Table: AM vs. Traditional Manufacturing for Liners
Vlastnosti | Výroba aditiv kovů (AM) | Traditional Manufacturing (Casting/Fabrication) |
---|---|---|
Složitost návrhu | Very High (Internal channels, lattices, optimized shapes) | Moderate to High (Limited by tooling, joining methods) |
Konsolidace částí | Excellent (Multiple parts integrated into one) | Limited (Requires welding, brazing, fasteners) |
Lead Time (Proto) | Short (Days/Weeks) | Long (Weeks/Months – requires tooling) |
Náklady na nástroje | None / Minimal | High (Molds, dies, jigs, fixtures) |
Materiálový odpad | Low (Near-net shape) | Moderate to High (Machining allowance, gating systems) |
Cooling Efficiency | Potentially Higher (Optimized, complex cooling schemes) | Good (Established methods, but geometrically limited) |
Odlehčení | Excellent (Topology optimization, lattices) | Omezený |
Ideal Volume | Low to Medium, High Complexity, Rapid Prototyping, Customization | High Volume, Simpler Designs |
Potential Failure | Process control variations (porosity, stress) | Weld/Brazing defects, Casting porosity/inclusions |
Export do archů
While AM presents compelling advantages, it’s crucial for engineers and procurement managers to partner with experienced metal AM service providers who possess deep expertise in process control, material science, and post-processing specific to demanding aerospace applications.
Material Focus: IN738LC and Hastelloy X for Extreme Environments
The selection of materials for jet engine combustion liners is paramount due to the incredibly harsh operating conditions: extreme temperatures (often exceeding 1500∘C flame temperature, with metal temperatures kept lower via cooling but still reaching 800−1100∘C), high pressures, oxidizing and corrosive environments, and severe thermal cycling. Nickel-based superalloys are the materials of choice for these demanding applications, offering an exceptional combination of high-temperature strength, creep resistance, fatigue life, and resistance to oxidation and corrosion.
For liners manufactured using metal additive manufacturing, two standout candidates are IN738LC (Inconel 738 Low Carbon) a Hastelloy X. These alloys possess properties that make them highly suitable for the layer-by-layer fusion processes inherent in AM, and their performance characteristics align perfectly with the requirements of a combustion liner. Sourcing high-quality, AM-optimized powders of these superalloys is critical for achieving the desired part density, microstructure, and mechanical properties.
Met3dp: Your Partner for High-Performance Superalloy Powders
At Met3dp, we understand the critical role materials play in additive manufacturing success. Leveraging our advanced powder production capabilities, including industry-leading gas atomization and Plasma Rotating Electrode Process (PREP) technologies, we produce high-sphericity, excellent-flowability metal powders specifically optimized for AM processes like SLM and EBM. Our expertise ensures that powders like IN738LC and Hastelloy X meet the stringent requirements of the aerospace industry for purity, particle size distribution, and morphology. Explore our range of metal powders and products.
IN738LC (Inconel 738 Low Carbon)
IN738LC is a precipitation-hardenable nickel-based superalloy renowned for its excellent high-temperature strength, creep resistance, and hot corrosion resistance, particularly in the intermediate temperature range (750−950∘C). The “LC” designation indicates low carbon content, which improves weldability and, importantly for AM, reduces the susceptibility to solidification cracking during the rapid heating and cooling cycles of the printing process.
- Key Properties & Benefits for Liners:
- High Creep Strength: Resists deformation under sustained load at elevated temperatures, crucial for maintaining liner shape and integrity over long operational periods.
- Excellent Hot Corrosion Resistance: Withstands attack from sulfur and other contaminants present in jet fuel combustion products.
- Good Oxidation Resistance: Forms a protective oxide layer to resist degradation in the high-temperature, oxygen-rich environment.
- Good Castability/Printability: The LC variant exhibits improved processing characteristics suitable for investment casting and, critically, for additive manufacturing processes like SLM.
- Precipitation Hardening: Achieves its high strength through heat treatment, which causes the precipitation of gamma prime (γ′) phases within the nickel matrix. Careful control of the AM process and post-build heat treatments is essential to achieve the desired microstructure and properties.
Hastelloy X
Hastelloy X is a solid-solution-strengthened nickel-chromium-iron-molybdenum superalloy. Unlike precipitation-hardened alloys like IN738LC, it derives its strength primarily from the alloying elements dissolved in the nickel matrix. It is known for its outstanding combination of high-temperature strength, oxidation resistance, and fabricability.
- Key Properties & Benefits for Liners:
- Výjimečná odolnost proti oxidaci: Offers superior resistance to oxidation up to very high temperatures (around 1200∘C), making it suitable for the hottest sections of the combustor.
- Good High-Temperature Strength: While generally not as strong as precipitation-hardened alloys at intermediate temperatures, it retains useful strength at very high temperatures.
- Excellent Formability and Weldability: Traditionally known for its ease of fabrication in wrought forms, these characteristics translate well to good processability in additive manufacturing. It is less prone to cracking compared to some other superalloys.
- Resistance to Stress Corrosion Cracking: Shows good resistance to cracking in chloride-bearing environments.
- Tepelná stabilita: Maintains its properties after long exposures to high temperatures.
Comparison Table: IN738LC vs. Hastelloy X for AM Liners
Vlastnictví | IN738LC | Hastelloy X | Relevance to Liners |
---|---|---|---|
Strengthening Mechanism | Precipitation Hardening (γ′) | Pevné posílení řešení | Affects heat treatment requirements and ultimate strength capabilities. |
Maximální teplota použití | Excellent up to ~980∘C | Excellent up to ~1200∘C (for oxidation) | Hastelloy X favored for the very hottest regions due to superior oxidation resistance. |
Pevnost v tahu | Very High (intermediate temps) | Good (especially at very high temps) | IN738LC often preferred where long-term creep resistance below 950∘C is critical. |
Odolnost proti oxidaci | Dobrý | Vynikající | Critical for longevity in the combustor environment. |
Hot Corrosion Resistance | Vynikající | Dobrý | Important for resisting fuel impurities. |
AM Processability | Good (LC variant helps) | Vynikající | Affects defect formation (cracking, porosity) during printing. |
Tepelné zpracování | Required (Solution + Aging) | Typically Solution Annealed | Impacts post-processing steps and final properties. |
Typické aplikace | Turbine blades, vanes, combustor components | Combustor liners, transition ducts, afterburners | Both are proven materials in hot sections of gas turbines. |
Export do archů
Why Met3dp Powders Matter:
The success of printing jet engine liners with IN738LC or Hastelloy X heavily relies on the quality of the starting metal powder. Met3dp ensures:
- High Sphericity & Flowability: Essential for uniform powder bed layering in SLM/EBM processes, leading to consistent melt pool behavior and high part density.
- Controlled Particle Size Distribution (PSD): Optimized PSD ensures good packing density and predictable melting characteristics.
- High Purity & Low Satellites: Minimizes contaminants and irregularities that can lead to defects in the final component.
- Batch Consistency: Strict quality control ensures reliable and repeatable results from batch to batch, crucial for aerospace qualification.
Choosing the right material, processed correctly using high-quality powder from a trusted supplier like Met3dp, is fundamental to leveraging the full potential of additive manufacturing for creating superior jet engine liners. Understanding the nuances between alloys like IN738LC and Hastelloy X allows engineers to select the optimal material based on the specific temperature profile and stress distribution within the liner design.
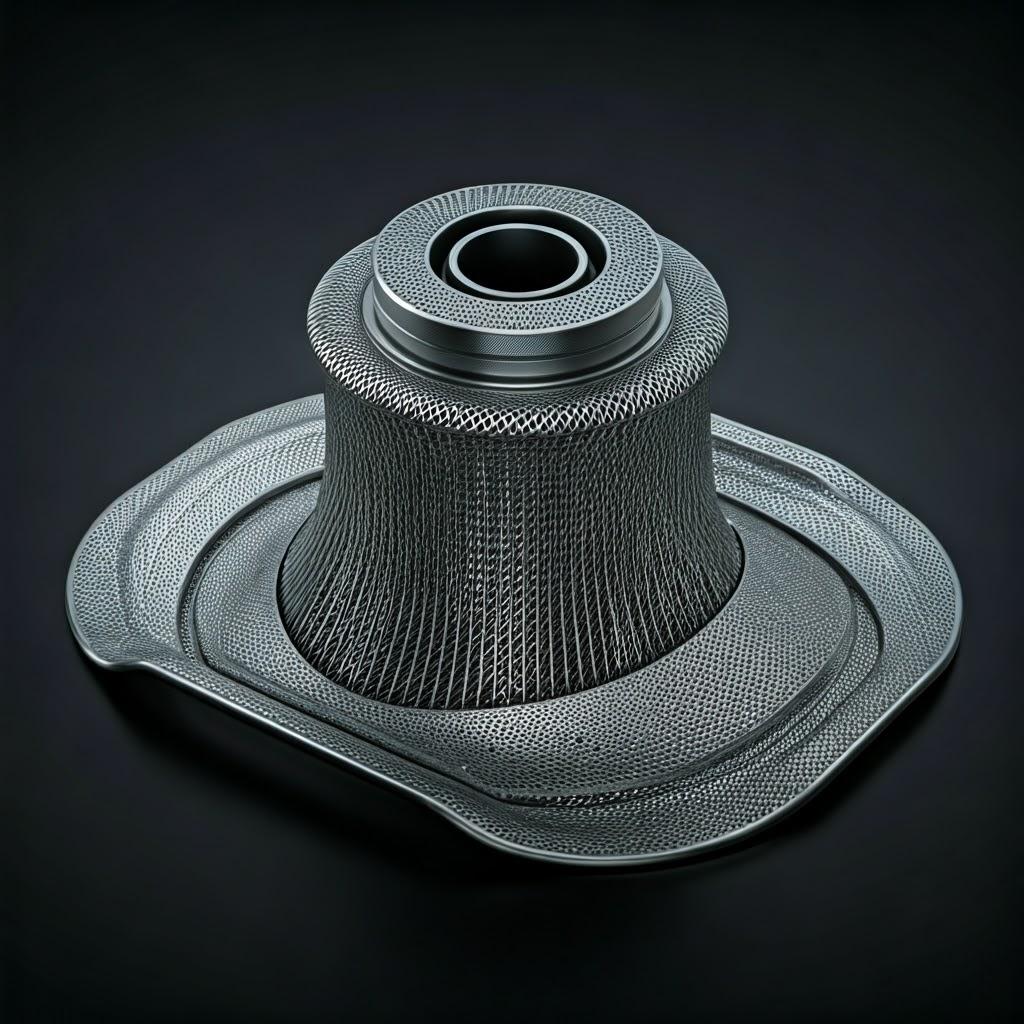
Design for Additive Manufacturing (DfAM): Optimizing Liner Geometry
Simply replicating a traditionally designed jet engine liner using additive manufacturing often fails to capture the full potential of the technology. To truly leverage the benefits of AM – lightweighting, improved cooling, part consolidation – engineers must embrace Design pro aditivní výrobu (DfAM). DfAM is a paradigm shift, moving beyond the constraints of conventional manufacturing and designing parts specifically optimized for the layer-by-layer AM process. For complex, high-value components like combustion liners operating in extreme environments, DfAM is not just beneficial; it’s essential for unlocking significant performance gains.
Key Principles of DfAM for Jet Engine Liners:
- Leveraging Geometric Freedom:
- Complex Cooling Channels: AM excels at creating conformal cooling channels that follow the contours of the liner walls, internal passages with optimized cross-sections (e.g., tear-drop shapes for better flow), and intricate networks that maximize heat transfer. This allows for more effective cooling using less bleed air, directly improving engine efficiency.
- Optimized Effusion Cooling: Instead of just drilling round holes, AM can create shaped holes (like fans or laid-back designs) oriented precisely to optimize the protective film of cooling air along the liner surface, enhancing cooling effectiveness and durability.
- Integrated Features: Brackets, bosses, mounting points, and other features that might have been separate, welded-on parts can be integrated directly into the liner design, reducing part count and eliminating potential failure points at joints.
- Topology Optimization and Lightweighting:
- Material Where Needed: Software tools analyze the stress distribution within the liner under operational loads. Topology optimization algorithms then remove material from low-stress regions, creating organic, load-path-optimized structures that are significantly lighter than their traditional counterparts while maintaining or even increasing stiffness and strength.
- Mřížové struktury: For areas requiring stiffness but not full density, internal lattice structures can be incorporated. These intricate networks significantly reduce weight and can also be designed to enhance heat dissipation or acoustic damping – functions relevant within a combustor environment.
- Konsolidace částí:
- Simplifying Assemblies: As mentioned earlier, AM allows multiple components of a traditional liner assembly (e.g., different sections, cooling rings, mounting flanges) to be redesigned and printed as a single, monolithic piece. This drastically reduces assembly time, eliminates joints prone to fatigue or leakage, and simplifies supply chain management for aerospace manufacturers and MRO providers.
- Considering Manufacturing Constraints (AM Specific):
- Podpůrné struktury: AM parts often require temporary support structures during the build process to anchor overhangs and dissipate heat. DfAM involves designing parts to minimize the need for supports, particularly in hard-to-reach internal areas. This includes orienting the part strategically on the build plate and using self-supporting angles (typically > 45 degrees from the horizontal) where possible. When supports are necessary, the design should ensure they are accessible for removal during post-processing.
- Orientace na stavbu: The orientation in which the liner is printed affects surface finish, the location and type of supports needed, residual stress accumulation, and potentially anisotropic material properties. DfAM considers the optimal orientation early in the design phase.
- Minimum Feature Size & Wall Thickness: AM processes have limitations on the smallest features (holes, slots) and thinnest walls they can reliably produce. Designers must adhere to process-specific guidelines, especially when working with challenging superalloys like IN738LC and Hastelloy X. Typical minimum wall thicknesses might range from 0.4mm to 1.0mm depending on the specific machine and material.
- Heat Dissipation: Large solid sections can accumulate heat during printing, potentially leading to stress and distortion. DfAM may involve designing internal voids or channels even if not strictly required for function, simply to manage thermal gradients during the build.
DfAM Workflow Considerations:
DfAM Step | Popis | Tools/Techniques | B2B/Supplier Relevance |
---|---|---|---|
Requirement Analysis | Define functional, thermal, structural, and lifetime requirements for the liner. | System Engineering, FEA (Initial) | Clear specs needed for quoting & manufacturing by AM service providers. |
Conceptual Design (AM) | Brainstorm designs leveraging AM freedom (complex cooling, lattices, integration). | CAD, Generative Design Software | Collaboration between OEM designers and AM experts (like Met3dp application engineers). |
Optimalizace topologie | Mathematically remove material from non-critical areas based on load cases. | FEA-based Optimization Software (e.g., Altair OptiStruct, Ansys Discovery) | Reduces material cost & weight, key selling points for wholesale components. |
Detailed Design | Refine geometry, add functional features, design support-minimizing features, define cooling hole patterns. | Advanced CAD, AM-specific modules | Manufacturability checks by AM supplier are crucial. |
Build Simulation | Predict thermal stresses, distortion, and potential build failures before printing. | AM Simulation Software (e.g., Ansys Additive Suite, Simufact Additive) | Reduces trial-and-error costs, ensures higher first-time build success rate. |
Support Strategy | Design necessary support structures for accessibility and minimal part scarring. | AM Preparation Software (e.g., Materialise Magics) | Experienced suppliers optimize support for quality and efficient removal. |
Post-Processing Plan | Define steps for heat treatment, support removal, machining, finishing based on design features. | Process Engineering | Integrated planning ensures final part meets all specifications. |
Export do archů
By integrating DfAM principles from the outset, engineers can design jet engine liners that are not only manufacturable via AM but are also lighter, more thermally efficient, and potentially more durable than their conventionally produced predecessors. Partnering with an AM provider knowledgeable in DfAM best practices for superalloys is key to realizing these advantages.
Achieving Precision: Tolerances, Surface Finish, and Dimensional Accuracy in AM Liners
While additive manufacturing offers incredible geometric freedom, achieving the tight tolerances, specific surface finishes, and high dimensional accuracy required for critical aerospace components like jet engine liners demands careful process control and often necessitates post-processing steps. Procurement managers and engineers must understand the typical capabilities and limitations of AM processes like Selective Laser Melting (SLM) and Electron Beam Melting (EBM) when specifying requirements for 3D printed liners.
Tolerances:
- General Achievable Tolerances: As-built tolerances for metal AM processes typically fall in the range of ±0.1mm to ±0.2mm (±0.004″ to ±0.008″) for smaller dimensions, and around ±0.1% to ±0.2% for larger dimensions. However, this can vary significantly based on:
- Part Size and Geometry: Larger parts and complex geometries are more prone to thermal distortion, affecting overall accuracy.
- Materiál: Superalloys like IN738LC and Hastelloy X can exhibit significant residual stress, impacting dimensional stability.
- Kalibrace stroje: Regular calibration and maintenance of the AM system are crucial.
- Orientace na stavbu: Orientation affects thermal gradients and support locations.
- Následné zpracování: Stress relief heat treatments can cause minor dimensional changes that need to be accounted for.
- Critical Tolerances: Features requiring tighter tolerances than the general as-built capability (e.g., mating surfaces, interfaces with other engine components, precise diameters for fuel nozzles or sensors) typically require post-machining (CNC milling, turning, grinding). It is essential to incorporate machining allowances into the DfAM stage for these features.
Povrchová úprava (drsnost):
- As-Built Surface Finish: The surface finish of as-built metal AM parts is inherently rougher than machined surfaces. Typical Ra (average roughness) values range from 6 µm to 20 µm (240 µin to 800 µin), influenced by:
- Tloušťka vrstvy: Thinner layers generally result in smoother surfaces but increase build time. Typical layer thicknesses are 30-60 µm.
- Velikost částic prášku: Finer powders can lead to smoother finishes but may affect flowability. Met3dp optimizes powder characteristics for a balance of printability and finish.
- Orientace: Surfaces parallel to the build plate (up-facing) tend to be smoother than vertical walls, which are smoother than down-facing surfaces that required support contact. Inclined surfaces exhibit the characteristic “stair-stepping” effect.
- Parametry procesu: Laser/electron beam power, scan speed, and strategy affect melt pool stability and surface characteristics.
- Zlepšení povrchové úpravy: For applications requiring smoother surfaces (e.g., for aerodynamic performance inside the liner or to reduce stress concentrations), various post-processing techniques are employed:
- Abrasive Blasting (Grit/Bead Blasting): Provides a uniform matte finish, removes loose powder, but doesn’t drastically reduce Ra.
- Tumbling/Vibratory Finishing: Uses media to smooth surfaces, effective for batches of smaller parts or accessible features.
- Chemical Polishing/Etching: Can smooth surfaces but requires careful control.
- Electropolishing: Provides a very smooth, bright finish by electrochemically removing material.
- Manual Polishing: Labor-intensive but used for specific critical areas.
- CNC obrábění: The most precise way to achieve a specific, smooth finish on functional surfaces.
Dimensional Accuracy & Quality Control:
- Ensuring Accuracy: Achieving consistent dimensional accuracy requires a holistic approach:
- Robust Process Control: Monitoring melt pool characteristics, chamber atmosphere, and thermal conditions during the build. High-quality AM systems with closed-loop control, like those potentially used by Met3dp partners or in-house, are advantageous.
- Simulation: Using simulation tools to predict and compensate for distortion.
- Optimized Support Strategy: Well-designed supports minimize warping during the build and prevent sagging.
- Controlled Post-Processing: Performing stress relief and HIP processes consistently.
- Inspection and Verification: Rigorous inspection is non-negotiable for aerospace components.
- Coordinate Measuring Machines (CMM): Used for precise measurement of critical dimensions and geometric dimensioning and tolerancing (GD&T) verification.
- 3D Scanning (Laser/Structured Light): Captures the overall geometry of the part, allowing comparison to the original CAD model and identification of deviations or warping.
- Nedestruktivní zkoušení (NDT): Methods like CT scanning are used not only for defect detection (see next section) but can also provide dimensional analysis of internal features.
Summary Table: Precision in AM Liners
Parametr | As-Built Capability (Typical) | Factors Influencing | Post-Processing Options for Improvement |
---|---|---|---|
Tolerance | ±0.1 to ±0.2 mm / ±0.1% to ±0.2% | Size, Geometry, Material, Machine | CNC obrábění |
Povrchová úprava | Ra 6-20 µm (240-800 µin) | Layer Thickness, Powder, Orientation | Machining, Polishing, Blasting, Tumbling |
Verification | CMM, 3D Scanning, NDT | Inspection Requirements, Criticality | N/A (Methods for checking accuracy) |
Export do archů
Engineers specifying AM liners must clearly define tolerance and surface finish requirements on drawings, indicating which apply “as-built” and which require post-processing. Collaborating with an experienced AM supplier is crucial to ensure these requirements are achievable and cost-effective.
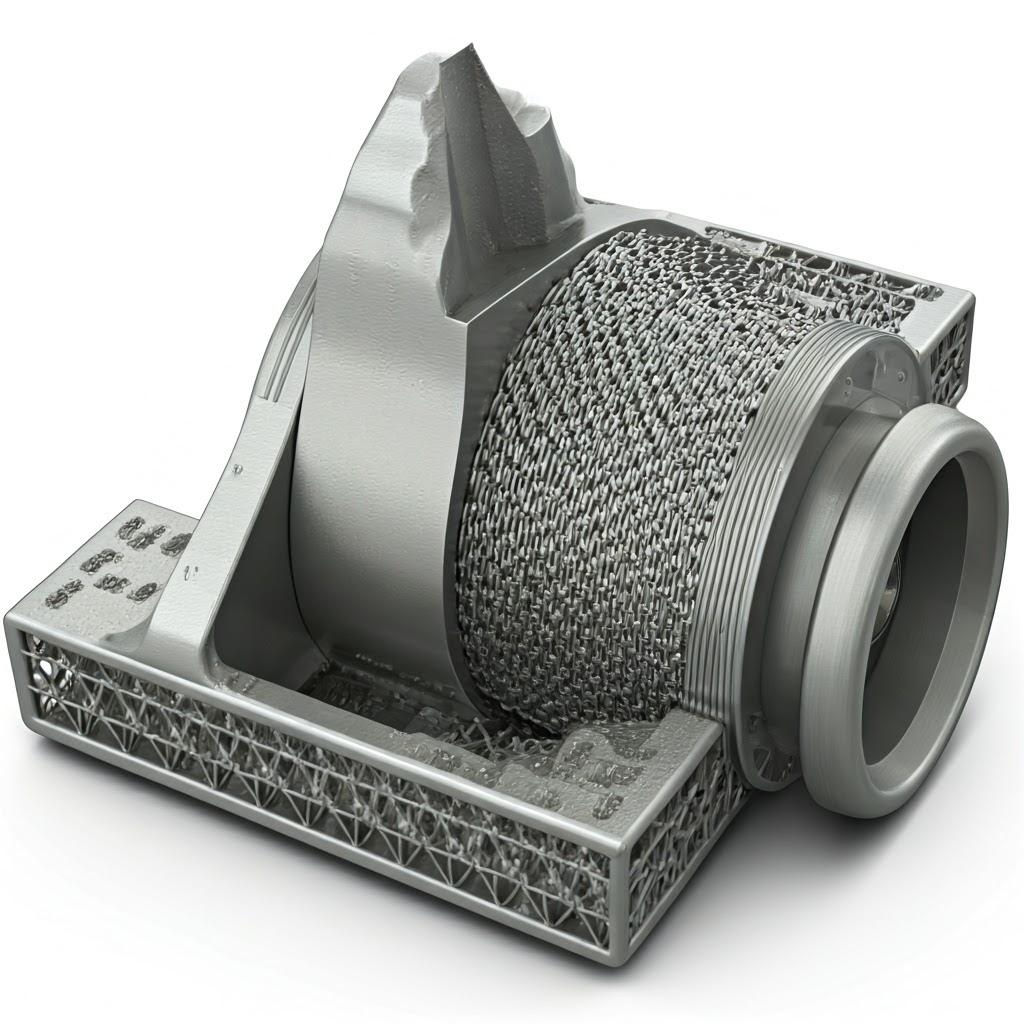
Beyond the Build: Essential Post-Processing for 3D Printed Liners
Printing the jet engine liner is only one part of the additive manufacturing workflow. For safety-critical aerospace components made from high-performance superalloys, post-processing steps are absolutely essential to achieve the required material properties, dimensional accuracy, surface finish, and overall part integrity. Skipping or improperly performing these steps can compromise the liner’s performance and durability.
Common Post-Processing Steps for AM Superalloy Liners:
- Stress Relief Heat Treatment:
- Účel: The rapid heating and cooling cycles inherent in powder bed fusion processes induce significant residual stresses within the printed part. These stresses can cause distortion upon removal from the build plate or even lead to cracking. Stress relief, typically performed while the part is still attached to the build plate in a controlled atmosphere furnace, reduces these internal stresses, improving dimensional stability and reducing crack susceptibility.
- Proces: Involves heating the part to a specific temperature (below the aging temperature for precipitation-hardened alloys like IN738LC), holding it for a period, and then slowly cooling it. Parameters depend heavily on the alloy and part geometry.
- Removal from Build Plate & Support Removal:
- Účel: Separating the part from the build plate and removing the temporary support structures.
- Metody: Often done using wire EDM (Electrical Discharge Machining) or a bandsaw to cut the part from the plate. Support removal can be challenging, especially for complex internal geometries. It may involve manual breaking/cutting for accessible supports, or CNC machining and potentially chemical etching for difficult-to-reach areas. Careful design (DfAM) minimizes support complexity.
- Izostatické lisování za tepla (HIP):
- Účel: HIP is a crucial step for improving the metallurgical integrity of AM aerospace parts. It uses high temperature (below melting point) and high inert gas pressure (typically Argon) applied uniformly to the part. This process effectively closes internal micro-porosity (like gas porosity or small lack-of-fusion defects) that can occur during printing. Eliminating porosity significantly improves fatigue life, ductility, and fracture toughness – properties critical for liner longevity under thermal cycling.
- Výhody: Results in a near-fully dense material, approaching the properties of wrought or cast equivalents. It is often a mandatory step for critical rotating or pressurized components in aerospace.
- Solution Annealing & Aging Heat Treatments (if applicable):
- Účel: To achieve the desired final microstructure and mechanical properties, particularly for precipitation-hardenable alloys like IN738LC.
- Proces:
- Žíhání roztoků: Heats the material to a high temperature to dissolve alloying elements and precipitates into a solid solution, followed by rapid cooling (quenching).
- Aging (Precipitation Hardening): Heats the part to an intermediate temperature for an extended period, causing the controlled precipitation of strengthening phases (like γ′ in IN738LC).
- Poznámka: For solid-solution strengthened alloys like Hastelloy X, a solution anneal might be performed after HIP or machining to optimize properties, but precipitation aging is not typically required. Precise control of temperatures, times, and atmospheres is critical.
- CNC obrábění:
- Účel: To achieve tight tolerances on critical features, create precise sealing surfaces, drill or ream specific holes, and attain required surface finishes that cannot be achieved “as-built” or through other finishing methods.
- Úvahy: Requires careful fixture design to hold the potentially complex AM part geometry. Machining allowances must be included in the DfAM phase. Understanding the potentially different machining behavior of AM materials compared to wrought is important.
- Povrchová úprava:
- Účel: To achieve the final required surface roughness, clean the part, or prepare it for subsequent coatings.
- Metody: As described previously (blasting, polishing, tumbling, etc.), chosen based on the specific requirements for different areas of the liner.
- Thermal Barrier Coatings (TBCs) (Optional but common):
- Účel: To further protect the liner material from the extreme combustion temperatures. TBCs are ceramic coatings (often Yttria-Stabilized Zirconia – YSZ) applied to the hot-gas-path surfaces. They provide thermal insulation, significantly reducing the metal temperature of the liner, allowing for higher gas temperatures or extending liner life.
- Použití: Typically applied using plasma spray or electron beam physical vapor deposition (EB-PVD) after final machining and surface preparation.
Workflow Integration:
The sequence and specific parameters of these post-processing steps are critical and must be carefully planned and executed. They represent a significant portion of the total manufacturing time and cost for an AM liner. Reputable metal AM service providers specializing in aerospace will have validated procedures and certified equipment for these critical post-processing operations. Understanding this full workflow is vital for accurate cost estimation and lead time planning by B2B procurement teams.
Navigating Challenges: Ensuring Quality in Additive Manufacturing of Liners
While metal AM offers transformative potential for jet engine liners, achieving consistent, high-quality results, especially with demanding superalloys, involves overcoming several inherent challenges. Awareness of these potential issues and partnering with knowledgeable providers who employ robust mitigation strategies is crucial for success in aerospace applications. Met3dp, through its focus on high-quality powders and deep understanding of AM processes, contributes significantly to overcoming these hurdles. Read more about our commitment and expertise on our O nás strana.
Common Challenges and Mitigation Strategies:
- Residual Stress and Distortion:
- Výzva: The rapid, localized heating and cooling during layer-wise fusion creates significant temperature gradients, leading to internal stresses. These stresses can cause parts to warp or distort during the build, after removal from the build plate, or during post-processing heat treatments. In severe cases, stress can lead to cracking.
- Mitigation:
- Optimalizované strategie skenování: Using techniques like island scanning, checkerboard patterns, or optimized vector lengths helps distribute heat more evenly.
- Build Plate Heating: Preheating the build plate reduces the thermal gradient between the molten material and the underlying layers/plate.
- Simulace procesu: Predicting stress accumulation and distortion allows for compensated designs or optimized build orientations and support strategies.
- Robustní podpůrné struktury: Adequate supports anchor the part firmly and help conduct heat away.
- Okamžitá úleva od stresu: Performing stress relief heat treatment directly after the build, often before support removal, is critical.
- Pórovitost:
- Výzva: Internal voids or pores within the printed material can act as stress concentrators, significantly degrading fatigue life and mechanical properties. Porosity can arise from trapped gas within the powder or melt pool (gas porosity) or incomplete fusion between layers or scan tracks (lack-of-fusion porosity).
- Mitigation:
- Vysoce kvalitní prášek: Using powder with low internal gas content, high sphericity, and controlled PSD (like those produced by Met3dp’s advanced atomization) is fundamental. Proper powder handling and storage to prevent moisture absorption are also key.
- Optimalizované parametry procesu: Fine-tuning laser/beam power, scan speed, layer thickness, and hatch spacing ensures complete melting and fusion.
- Controlled Atmosphere: Maintaining a high-purity inert gas atmosphere (Argon or Nitrogen) in the build chamber minimizes oxidation and gas pickup.
- Izostatické lisování za tepla (HIP): As discussed, HIP is highly effective at closing internal porosity, making it a standard step for critical aerospace parts.
- Cracking:
- Výzva: Superalloys, particularly precipitation-hardenable ones like IN738LC, can be susceptible to solidification cracking (during cooling of the melt pool) or liquation cracking (re-melting of lower-melting-point phases in grain boundaries) due to thermal stresses.
- Mitigation:
- Alloy Selection/Modification: Using variants designed for better AM processability (e.g., IN738LC vs. standard IN738).
- Parameter Optimization: Controlling energy input and cooling rates to minimize thermal stresses.
- Scan Strategy Optimization: Reducing residual stress buildup.
- Appropriate Heat Treatments: Carefully controlled stress relief and homogenization treatments.
- Odstranění podpůrné konstrukce:
- Výzva: Removing supports, especially dense or internal ones within complex liner geometries, can be difficult, time-consuming, and risk damaging the part surface.
- Mitigation:
- DfAM: Designing parts to be self-supporting where possible, using optimized support types (e.g., conical, thin-walled, perforated) that are easier to remove.
- Strategic Orientation: Choosing a build orientation that minimizes critical supports or places them in accessible locations.
- Advanced Removal Techniques: Employing CNC machining, EDM, or electrochemical methods when manual removal is impractical or risks damage.
- Material Property Consistency & Anisotropy:
- Výzva: Mechanical properties (strength, ductility, fatigue life) can sometimes vary depending on the build direction (anisotropy) due to the layered microstructure and thermal history. Ensuring consistent properties throughout the part and from build to build is crucial.
- Mitigation:
- Process Parameter Control: Maintaining tight control over all printing parameters.
- Tepelný management: Using build plate heating and controlled cooling.
- Post-Processing (HIP & Heat Treatment): HIP and appropriate heat treatments help homogenize the microstructure and reduce anisotropy, leading to more uniform properties.
- Rigorous Testing: Extensive material testing (tensile, fatigue, creep) on witness coupons built alongside the parts, often tested in different orientations.
- Nedestruktivní zkoušení (NDT):
- Výzva: Reliably detecting critical internal defects (pores, cracks, lack of fusion) within complex AM geometries requires sophisticated NDT techniques.
- Mitigation:
- Computed Tomography (CT) Scanning: Provides a full 3D view of the internal structure, highly effective for detecting volumetric defects and verifying internal channel geometry.
- Other Methods: Depending on the part and defect type, techniques like fluorescent penetrant inspection (FPI) for surface cracks, ultrasonic testing (UT), or radiography might be employed, though CT scanning is increasingly preferred for complex AM aerospace parts.
Successfully manufacturing high-quality jet engine liners via AM requires deep expertise across materials science, process engineering, DfAM, simulation, post-processing, and quality control. Partnering with a vertically integrated solutions provider or a specialized AM service bureau with a proven track record in aerospace superalloys is paramount for mitigating these challenges and ensuring part conformity and reliability.
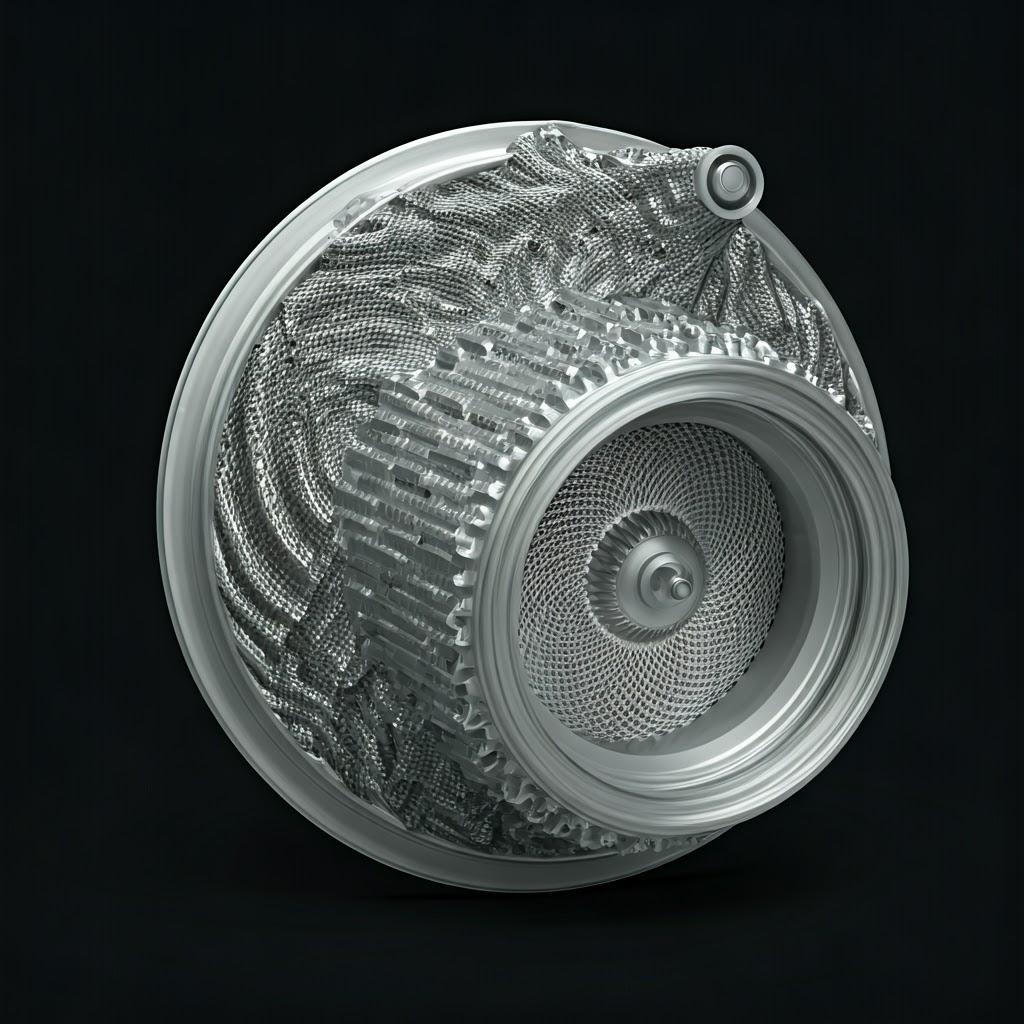
Supplier Selection: Choosing the Right Metal AM Partner for Aerospace Components
Selecting the right manufacturing partner is a critical decision when sourcing additively manufactured jet engine liners. Given the stringent requirements of the aerospace industry, the complexity of the components, and the advanced nature of the materials and processes involved, simply choosing the provider with the lowest quote is rarely the best strategy. Procurement managers and engineering teams need to evaluate potential metal additive manufacturing service providers based on a comprehensive set of criteria to ensure quality, reliability, and compliance.
Key Criteria for Evaluating Metal AM Suppliers for Aerospace Liners:
- Aerospace Certifications:
- AS9100: This is the standard quality management system (QMS) requirement for the aviation, space, and defense industries. Ensure the supplier holds a current AS9100 certification.
- NADCAP: National Aerospace and Defense Contractors Accreditation Program. While AS9100 covers the QMS, NADCAP provides specific accreditation for special processes like heat treatment, non-destructive testing (NDT), welding (less relevant for monolithic AM parts but indicative of process control), and potentially specific AM technologies as standards evolve. Look for NADCAP accreditation for relevant post-processing steps.
- OEM Approvals: Specific engine manufacturers (e.g., GE, Rolls-Royce, Pratt & Whitney) often have their own supplier approval processes and material/process specifications. Verify if the supplier is approved by the relevant OEMs if required.
- Proven Experience with Superalloys:
- Materiálové znalosti: The supplier must demonstrate extensive experience printing and post-processing the specific superalloys required (e.g., IN738LC, Hastelloy X). Ask for case studies, examples of similar parts produced, and material property data generated from their processes.
- Parameter Development: They should have well-developed and validated process parameters for the specific alloy-machine combination to ensure optimal density, microstructure, and mechanical properties.
- Technology and Equipment:
- Vhodná technologie AM: Verify they operate the right type of powder bed fusion system (SLM or EBM) suitable for the application and material. Understand the specific machine models they use and their capabilities (build volume, laser/beam power, monitoring systems).
- Machine Maintenance & Calibration: Ensure they have rigorous procedures for machine maintenance and calibration to guarantee consistent performance.
- Post-Processing Capabilities: Evaluate their in-house or tightly controlled outsourced capabilities for critical post-processing steps like stress relief, HIP, multi-axis CNC machining, NDT (especially CT scanning), and potentially TBC application. In-house capabilities often offer better control and shorter lead times.
- Material Traceability and Handling:
- Powder Sourcing: Understand where they source their metal powders. Do they partner with reputable powder manufacturers known for aerospace-grade quality, like Met3dp, or produce their own? High-quality input materials are non-negotiable.
- Powder Management: Strict procedures for powder handling, storage (controlling humidity), sieving, recycling (if applicable), and batch tracking are crucial to prevent contamination and ensure consistency. Full traceability from raw powder batch to final part is essential.
- Quality Management System (QMS) & Documentation:
- Robust QMS: Beyond AS9100, assess the depth and implementation of their QMS. This includes process control documentation, operator training records, inspection procedures, non-conformance handling, and corrective action processes.
- Comprehensive Documentation: Aerospace requires extensive documentation, including certificates of conformance, material certifications, process logs, inspection reports, and NDT results. Ensure the supplier can provide the required documentation package.
- Engineering and Technical Support:
- DfAM Expertise: Can they provide guidance on optimizing the liner design for additive manufacturing?
- Simulační schopnosti: Do they use build simulation software to predict and mitigate risks?
- Application Engineering: Do they have engineers who understand the functional requirements of aerospace components and can collaborate effectively? Companies like Met3dp, offering comprehensive solutions, often provide strong application development services.
- Řízení projektů a komunikace:
- Clear Communication: Look for responsive communication, clear points of contact, and proactive updates.
- Project Management: Ensure they have systems in place to manage complex projects, track progress, and meet agreed-upon deadlines.
Evaluation Checklist Summary:
Kritéria | Key Questions to Ask | Importance (Aerospace) |
---|---|---|
Certifikace | AS9100? NADCAP (Heat Treat, NDT)? OEM Approvals? | Mandatory |
Superalloy Experience | Which specific alloys? Similar parts produced? Material data available? Parameter validation? | Velmi vysoká |
Technology/Equipment | Right AM process (SLM/EBM)? Machine specs? In-house Post-Processing (HIP, CNC, NDT)? Calibration procedures? | Velmi vysoká |
Material Traceability | Powder source (Quality supplier like Met3dp?) Handling/Storage/Recycling procedures? Batch tracking? | Mandatory |
QMS & Documentation | Depth of QMS? Process control? Inspection methods? Required documentation provided (CoC, Material Certs, NDT reports)? | Mandatory |
Technická podpora | DfAM guidance? Simulation capabilities? Application understanding? | Vysoký |
Project Mgmt/Communication | Responsiveness? Point of contact? Progress tracking? On-time delivery record? | Vysoký |
Export do archů
Choosing a supplier is about building a partnership, especially for critical components. A thorough evaluation based on these criteria will help ensure you select a metal AM provider capable of delivering high-quality, compliant jet engine liners reliably.
Understanding Costs and Timelines for 3D Printed Jet Engine Liners
While the performance benefits of using additive manufacturing for jet engine liners are compelling, understanding the associated costs and typical lead times is crucial for project planning and budgeting by procurement professionals and engineering managers. The cost structure for AM parts differs significantly from traditional manufacturing, and several factors influence the final price and delivery schedule.
Key Cost Drivers for AM Jet Engine Liners:
- Náklady na materiál:
- Superslitiny: Nickel-based superalloys like IN738LC and Hastelloy X are inherently expensive materials. The cost is driven by the price of the raw elements (Nickel, Chromium, Cobalt, Molybdenum, etc.) and the sophisticated atomization process required to produce high-quality, spherical powder suitable for AM. The amount of powder consumed (part weight + supports + potential waste) is a major cost factor. Sourcing from reputable suppliers ensures quality but comes at a premium price point necessary for aerospace-grade materials.
- AM Machine Time:
- Doba výstavby: This is often the largest single cost component. Build time depends on:
- Část Objem: Larger parts naturally take longer.
- Part Height: Build time is directly related to the number of layers required (height divided by layer thickness). Tall parts take longer than flat parts of the same volume.
- Complexity & Supports: Intricate features and extensive support structures increase the area to be scanned by the laser/electron beam, adding time.
- Nesting: Printing multiple parts simultaneously on one build plate can amortize setup time but may increase overall build duration.
- Machine Depreciation & Operating Costs: The capital cost of industrial metal AM systems is high, and this is factored into hourly rates, along with energy consumption, inert gas usage, filters, etc.
- Doba výstavby: This is often the largest single cost component. Build time depends on:
- Náklady na pracovní sílu:
- Nastavení a demontáž: Preparing the machine, loading powder, removing the build, and initial cleaning require skilled technician time.
- Následné zpracování: This can be very labor-intensive. Manual support removal, detailed inspection, fixture setup for machining, manual polishing, and NDT interpretation all contribute significantly to labor costs.
- Engineering & QA: DfAM support, simulation, quality checks, and documentation preparation also represent labor costs.
- Post-Processing Intensity:
- Mandatory Steps: Stress relief and HIP are generally required for liners and add fixed costs per batch.
- Obrábění: The amount of CNC machining required for tight tolerances or specific finishes significantly impacts cost. Multi-axis machining of complex AM shapes can be expensive.
- Povrchová úprava: Depending on the method (blasting vs. polishing) and the extent required.
- Povlaky: Application of TBCs is an additional, often substantial, cost.
- Nedestruktivní zkoušení (NDT):
- CT Scanning: While providing invaluable data, CT scanning equipment is expensive to own and operate, adding to the per-part cost. Other NDT methods also incur costs.
- Quality Assurance & Documentation:
- The rigorous inspection, testing, and documentation required for flight-critical aerospace components contribute to the overall cost.
- Objem objednávky:
- Úspory z rozsahu: While AM avoids tooling costs, some economies of scale exist. Setup costs are amortized over larger batches. Optimized nesting on build plates can improve machine utilization. However, the cost reduction per part with volume is typically less dramatic than in high-volume traditional processes like casting.
Typical Lead Times:
Lead times for AM liners can vary widely but generally offer advantages over traditional methods, especially for prototypes and low-volume production.
- Prototyping/First Article: Obvykle se pohybuje od 2 to 8 weeks. This includes DfAM review, build simulation (optional but recommended), printing, all post-processing steps (including potential delays for external HIP or heat treatment), inspection, and documentation. Design complexity and post-processing requirements are major drivers.
- Series Production: Once the process is fully qualified, lead times for subsequent batches can be optimized but remain dependent on build time, post-processing workflow, and batch size. Predictable lead times might be established based on contractual agreements with the 3D tisk z kovu supplier. Supply chain factors (powder availability, post-processing queue times) can influence consistency.
Cost vs. Traditional Methods:
- Direct Cost: For simpler liner designs produced in high volumes, traditional fabrication or casting may still have a lower direct per-part cost.
- Total Cost of Ownership: AM becomes more competitive when considering:
- High Complexity: Parts with intricate cooling or geometry impossible/expensive traditionally.
- Konsolidace částí: Reducing assembly labor and improving reliability.
- Zkrácení doby realizace: Faster development cycles, quicker replacement parts.
- Vylepšený výkon: Lightweighting and better cooling leading to fuel savings or longer component life (value proposition).
- No Tooling: Significant savings for low-volume or prototype parts.
Procurement managers should request detailed quotes breaking down these cost elements and work with suppliers to optimize designs and processes for cost-effectiveness without compromising quality or performance.
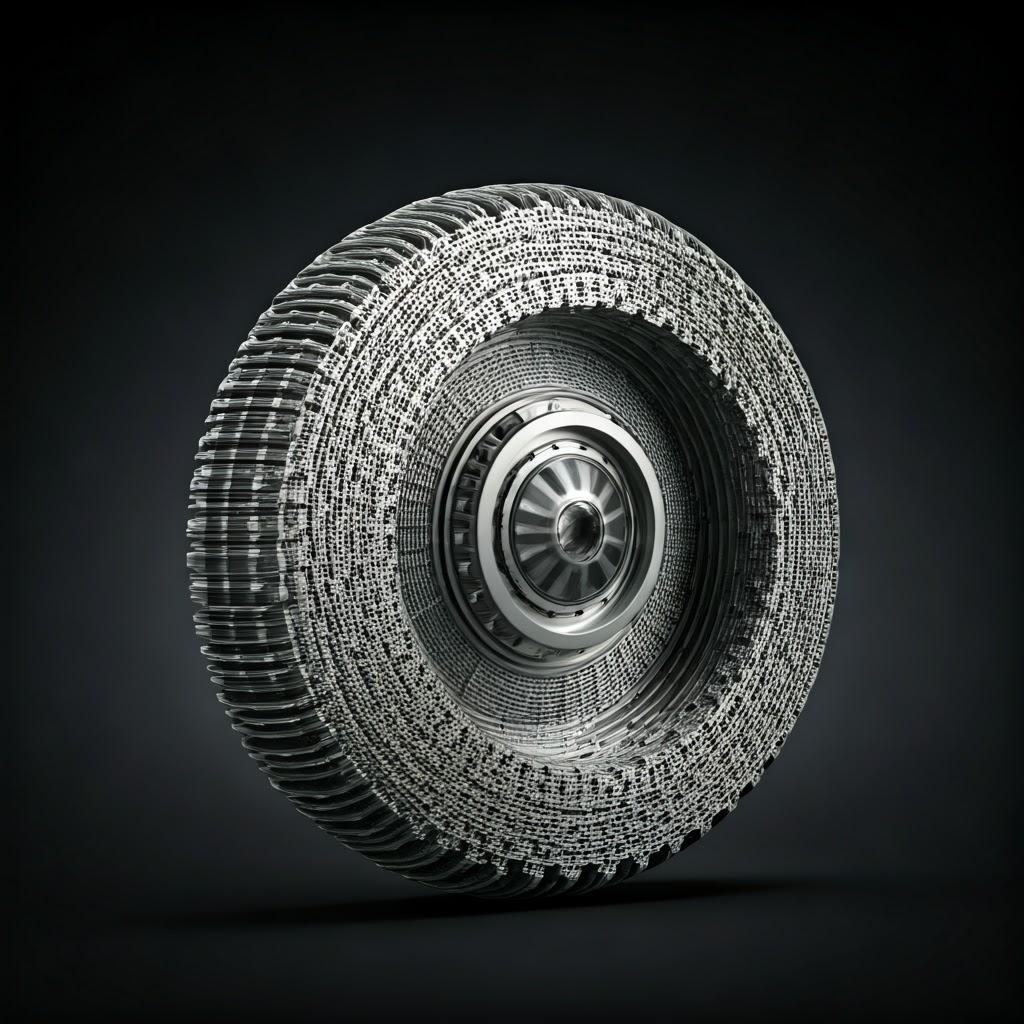
Frequently Asked Questions (FAQ) about 3D Printed Jet Engine Liners
Q1: How does the lifespan and durability of a 3D printed jet engine liner compare to a traditionally manufactured one (e.g., cast or fabricated)?
A1: The goal of using additive manufacturing for jet engine liners is often to match or exceed the lifespan and durability of traditional parts. AM allows for the use of advanced superalloys like IN738LC and Hastelloy X, processed to near full density via techniques like SLM/EBM combined with HIP. Furthermore, the design freedom offered by AM enables the creation of superior cooling schemes (complex channels, optimized effusion holes) that can significantly lower the operating metal temperature of the liner. Lower metal temperatures directly translate to reduced thermal fatigue, creep, and oxidation, potentially leading to a longer component life. However, achieving this requires rigorous process control, thorough NDT, and extensive qualification testing (including fatigue, creep, and engine endurance tests) to validate the performance and ensure it meets or exceeds the requirements set for traditionally manufactured liners. Early applications have shown promising results, but qualification is key for each specific design and application.
Q2: Is 3D printing jet engine liners cost-competitive with traditional methods like investment casting or sheet metal fabrication?
A2: The cost-competitiveness depends heavily on several factors. For highly complex liner designs incorporating intricate cooling features, topology optimization for lightweighting, or part consolidation (reducing multiple fabricated pieces to one), AM can be very cost-competitive or even cheaper when considering the total cost of ownership. This is because traditional methods would struggle or find it prohibitively expensive to create such complex features, and assembly costs are reduced. For simpler designs or very high production volumes, traditional methods like casting (after initial tooling investment) or sheet metal fabrication might still offer a lower per-part cost. However, AM offers significant savings by eliminating tooling costs (especially beneficial for prototypes and low volumes) and drastically reducing lead times for development and replacement parts. The value proposition for AM liners often lies in performance improvements (efficiency, weight) and accelerated innovation cycles rather than solely on direct per-part cost comparison for simple, high-volume components.
Q3: What specific certifications and qualifications are typically required for flight-critical additive manufacturing parts like combustion liners?
A3: Manufacturing flight-critical aerospace components via AM requires adherence to stringent certification and qualification protocols. Key requirements include:
- Quality Management System: The manufacturer must operate under an AS9100 certified QMS.
- Special Process Accreditations: NADCAP accreditation is typically required for critical post-processing steps such as heat treatment, NDT, and potentially welding (if any joining is involved, though AM aims to minimize this). As AM matures, specific NADCAP checklists for AM processes are becoming more common.
- Specifikace materiálu: The specific metal powder (e.g., IN738LC, Hastelloy X) must meet aerospace material specifications (AMS standards) regarding composition, powder characteristics, and traceability.
- Process Specification: The entire manufacturing process (printing parameters, build orientation, support strategy, post-processing steps including HIP, heat treatment, machining) must be rigorously defined, documented, and locked down in a process specification.
- Part Qualification: Extensive testing is required on parts produced using the defined process. This includes dimensional verification, NDT (often 100% CT scanning), destructive testing (tensile, fatigue, creep tests on witness coupons or sacrificial features), microstructural analysis, and potentially rig or full engine testing to demonstrate the part meets all performance and durability requirements.
- OEM Requirements: Major engine manufacturers often have their own specific requirements documents that suppliers must adhere to, potentially including approved supplier lists for materials and processes.
Meeting these requirements involves significant investment in process control, quality systems, testing, and documentation.
Conclusion: The Future is Additive – Advancing Jet Engine Technology with 3D Printing
The journey through the intricacies of manufacturing jet engine liners using metal additive manufacturing reveals a technology that is not just a novelty but a powerful enabler for the future of aerospace propulsion. The ability to overcome the limitations of traditional methods opens doors to unprecedented levels of design complexity, particularly in creating highly optimized cooling systems crucial for boosting engine efficiency and reducing emissions. By leveraging AM, engineers can consolidate parts, significantly reduce weight through topology optimization and lattice structures, and drastically shorten development cycles, accelerating the pace of innovation.
The successful implementation of AM for critical components like liners hinges on mastering several key areas: employing Design for Additive Manufacturing (DfAM) principles, selecting the right high-performance superalloys like IN738LC and Hastelloy X, utilizing high-quality metal powders, precisely controlling the printing process, and diligently executing essential post-processing steps such as HIP and heat treatments. Overcoming challenges related to residual stress, porosity, and achieving stringent tolerances requires deep expertise and robust quality control.
Choosing the right manufacturing partner – one with proven aerospace certifications, deep material and process knowledge, comprehensive capabilities, and a commitment to quality – is paramount. Companies like Met3dp, with their foundation in advanced powder manufacturing using gas atomization and PREP technologies, and a vision towards providing comprehensive solutions spanning materials, equipment, and application development, are playing a vital role in this ecosystem. We empower aerospace manufacturers to harness the full potential of metal AM.
As the aerospace industry continues its relentless push towards more sustainable, efficient, and higher-performing aircraft, metal additive manufacturing will undoubtedly play an increasingly central role. For components like jet engine liners operating at the heart of the engine’s power, AM offers a clear pathway to achieving next-generation performance goals. The future of jet engine technology is undeniably intertwined with the advancements and adoption of additive manufacturing.
Sdílet na
MET3DP Technology Co., LTD je předním poskytovatelem řešení aditivní výroby se sídlem v Qingdao v Číně. Naše společnost se specializuje na zařízení pro 3D tisk a vysoce výkonné kovové prášky pro průmyslové aplikace.
Dotaz k získání nejlepší ceny a přizpůsobeného řešení pro vaše podnikání!
Související články
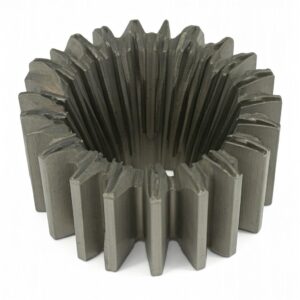
Vysoce výkonné segmenty lopatek trysek: Revoluce v účinnosti turbín díky 3D tisku z kovu
Přečtěte si více "O Met3DP
Nedávná aktualizace
Náš produkt
KONTAKTUJTE NÁS
Nějaké otázky? Pošlete nám zprávu hned teď! Po obdržení vaší zprávy obsloužíme vaši žádost s celým týmem.
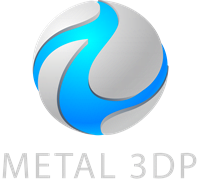
Kovové prášky pro 3D tisk a aditivní výrobu
SPOLEČNOST
PRODUKT
kontaktní informace
- Město Qingdao, Shandong, Čína
- [email protected]
- [email protected]
- +86 19116340731