Custom Bone Plates via Titanium 3D Printing
Inhaltsübersicht
Custom Bone Plates via Titanium 3D Printing: A New Era in Orthopedic Solutions
The field of orthopedic surgery is undergoing a profound transformation, driven by advancements in materials science and manufacturing technology. Among the most exciting developments is the application of metal additive manufacturing, commonly known as 3D-Druck, to create patient-specific medical implants. This is particularly impactful in the realm of non-load-bearing bone plates, where customization offers significant advantages. Utilizing materials like Ti-6Al-4V ELI (Extra Low Interstitials) titanium alloy, metal 3D printing enables the production of custom bone plates with intricate geometries that perfectly match a patient’s unique anatomy. This technology moves beyond the limitations of traditional, one-size-fits-many implants, paving the way for improved surgical outcomes, reduced operating times, and enhanced patient recovery, especially in complex craniofacial, maxillofacial, and pediatric applications. For medical device suppliers, distributors, and hospital procurement departments, understanding the capabilities and nuances of this technology is crucial for staying at the forefront of orthopedic care. Companies like Met3dp are pioneering these advancements, offering comprehensive solutions from high-quality metal powders to advanced printing systems, empowering the medical industry to embrace personalized medicine.
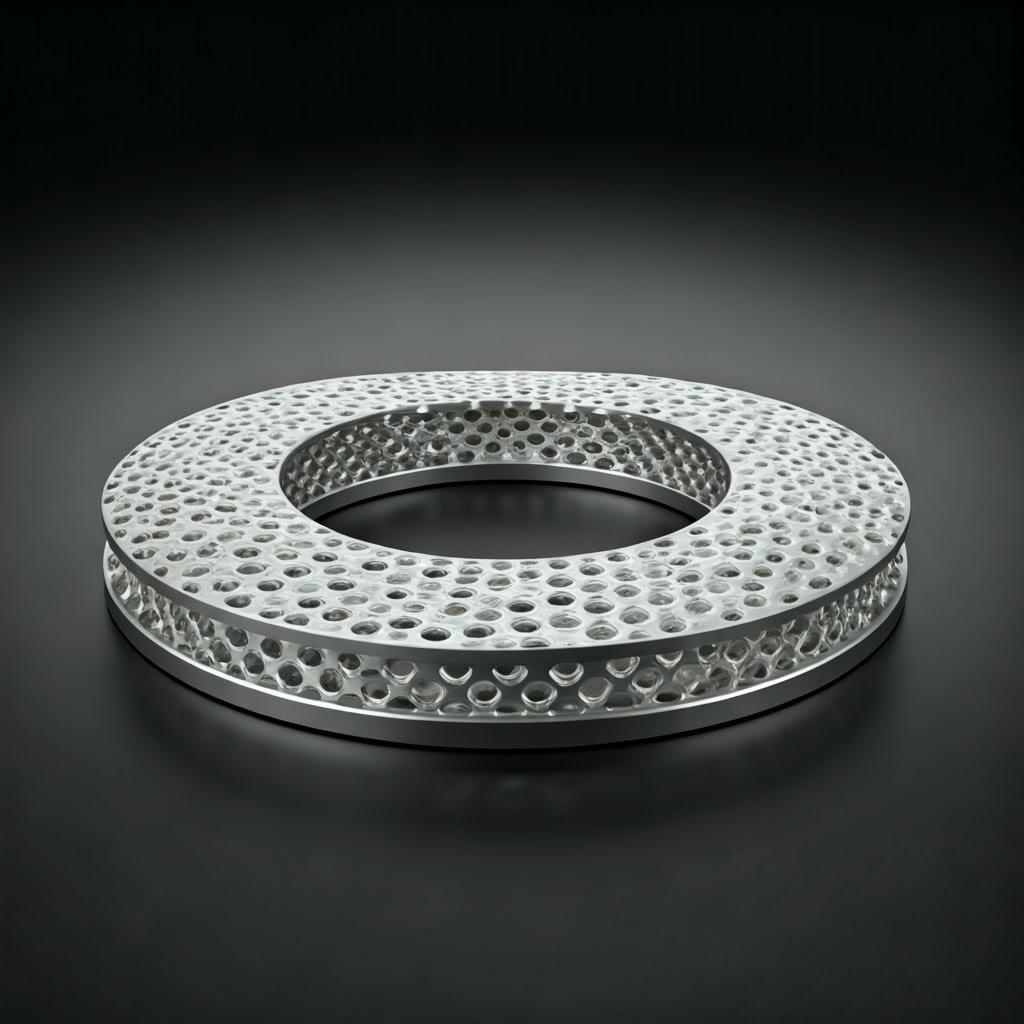
Introduction: Revolutionizing Orthopedics with Custom Titanium Bone Plates
Orthopedic surgery has long relied on bone plates to stabilize fractures, fuse joints, and reconstruct skeletal defects. Traditionally, these plates have been manufactured using subtractive methods, typically machining standard shapes and sizes from blocks of medical-grade metal. While effective for many common fractures, this approach often falls short when dealing with complex anatomies or unique patient needs, particularly in non-load-bearing situations where precise fit and contouring are paramount for optimal function and aesthetics. Non-load-bearing plates are typically used in areas like the skull, face, or in certain pediatric cases where the mechanical stresses are relatively low, but the need for anatomical accuracy is high.
The advent of metal additive manufacturing (AM), or 3D printing, represents a paradigm shift in the design and production of these critical medical devices. Instead of removing material, AM builds components layer by layer directly from a digital model, typically derived from a patient’s CT or MRI scan. This process unlocks unprecedented design freedom, allowing surgeons and biomedical engineers to create bone plates that are truly patient-specific.
The Power of Personalization:
Imagine a patient requiring craniofacial reconstruction after trauma. Using traditional methods might involve manually bending a standard plate during surgery – a time-consuming process that relies heavily on the surgeon’s skill and may result in a suboptimal fit. With metal 3D printing, a plate can be designed virtually, perfectly mirroring the contours of the patient’s skull, incorporating necessary screw holes in ideal locations, and even integrating features like porous structures to encourage bone ingrowth (osseointegration).
Material Excellence: The Role of Titanium:
Titanium alloys, particularly Ti-6Al-4V ELI, are the materials of choice for these applications. This specific grade offers an exceptional combination of:
- Biokompatibilität: It is well-tolerated by the human body, minimizing the risk of adverse reactions.
- Korrosionsbeständigkeit: It withstands the harsh environment within the body without degrading.
- Verhältnis Stärke/Gewicht: It provides necessary stability without excessive bulk or weight.
- Osseointegrationspotenzial: Surface modifications and porous structures possible with AM can enhance bone integration.
- MRI/CT Compatibility: It generally causes less artifact interference in medical imaging compared to some other metals.
The ‘ELI’ designation signifies Extra Low Interstitials (oxygen, nitrogen, carbon, iron), which enhances the material’s ductility and fracture toughness, crucial properties for implant safety and longevity.
Additive Manufacturing Technologies:
Several AM processes can be used for titanium bone plates, with Powder Bed Fusion (PBF) methods being the most common:
- Selective Laser Melting (SLM) / Direct Metal Laser Sintering (DMLS): Uses a high-power laser to selectively fuse regions of a metal powder bed.
- Selektives Elektronenstrahlschmelzen (SEBM): Uses an electron beam in a vacuum environment to melt the powder. SEBM, like the systems developed by Met3dp, often results in lower residual stresses and can process certain materials more effectively, making it highly suitable for medical implant production.
Impact on Healthcare:
The implications of custom 3D printed bone plates are far-reaching:
- Improved Surgical Outcomes: Better anatomical fit leads to more stable fixation, potentially faster healing, and improved functional and aesthetic results.
- Reduced Operating Time: Pre-contoured, patient-specific plates eliminate the need for intraoperative bending, saving valuable time in the operating room.
- Complex Case Solutions: Enables effective treatment for challenging cases with unique anatomical variations or significant bone loss.
- Optimierung der Lieferkette: Potential for on-demand manufacturing reduces the need for hospitals and distributors to maintain large inventories of standard plates in various sizes.
As a leader in metal additive manufacturing, Met3dp provides not only state-of-the-art SEBM printing equipment but also meticulously engineered Metallpulver like Ti-6Al-4V ELI, ensuring the highest quality and consistency required for critical medical applications. Our deep expertise in both materials science and AM processes positions us as a key partner for medical device manufacturers, wholesale suppliers, and research institutions looking to leverage the transformative power of 3D printing in orthopedics. The journey towards personalized, high-performance orthopedic implants is accelerating, and custom titanium bone plates are at the vanguard of this revolution.
What are Non-Load-Bearing Bone Plates Used For? Applications and Indications
While load-bearing implants like hip stems or spinal fusion cages garner significant attention, non-load-bearing bone plates play an equally vital role in restoring form and function in specific anatomical regions. These plates are designed for fixation in areas where the primary forces are relatively low, and the main goals are anatomical reduction, stabilization for healing, and restoration of contour. The ability to customize these plates using metal 3D printing offers profound benefits in these often complex and delicate surgical fields. Understanding the specific applications is key for surgeons, medical device designers, and procurement specialists in hospitals and distribution networks.
Key Application Areas:
- Craniofacial Surgery: This is perhaps the most prominent area for custom non-load-bearing plates.
- Cranioplasty: Repairing defects in the skull resulting from trauma, tumor resection, or decompressive craniectomy. A precise fit is crucial for protecting the brain and achieving good cosmetic outcomes. 3D printing allows for large, complex plates that perfectly match the contours of the patient’s skull defect, often incorporating features like integrated flanges for screw fixation or porous surfaces to encourage tissue integration.
- Orbital Floor Reconstruction: Repairing fractures of the thin bones surrounding the eye socket. Accuracy is critical to restore eye position and movement and prevent complications like double vision (diplopia) or sunken eye (enophthalmos). Custom plates can precisely replicate the complex 3D shape of the orbital floor.
- Mandibular (Jaw) Reconstruction: While the mandible experiences significant forces during chewing, certain reconstructive plates, especially those used for bridging defects after tumor removal or trauma in specific locations, or for guiding bone regeneration, may be primarily non-load-bearing or designed for temporary stabilization before definitive load-bearing reconstruction. Customization ensures proper alignment and contour.
- Midface Reconstruction (e.g., Le Fort fractures): Stabilizing complex fractures of the upper jaw and surrounding facial bones. Anatomical reduction is key for restoring facial structure and occlusion (bite).
- Maxillofacial Surgery: Overlapping significantly with craniofacial surgery, this specifically includes procedures involving the jaws and face.
- Orthognathic Surgery: Corrective jaw surgery to address malocclusion and facial asymmetry. While fixation needs to be stable, the plates used are often relatively small and contoured to fit the repositioned bone segments. Custom guides and plates can enhance precision.
- Zygomatic (Cheekbone) Fractures: Restoring the prominence and symmetry of the cheekbone.
- Neurochirurgie: Primarily for cranioplasty applications, ensuring seamless integration with neurosurgical procedures. The ability to create thin yet strong plates that conform perfectly to the skull defect is highly advantageous.
- Pediatric Orthopedics: Children’s bones are still growing, and anatomical variations are common.
- Corrective Osteotomies: Surgical cuts in bone to correct deformities (e.g., in the upper limbs or skull). In certain low-stress applications, custom plates can provide stable fixation tailored to the child’s unique anatomy without interfering with growth plates if designed carefully.
- Congenital Deformity Correction: Addressing skeletal abnormalities present at birth, particularly in the craniofacial region.
- Hand and Foot Surgery: Fixation of small bone fractures or fusions in the fingers, wrist, toes, and foot. While these bones experience forces, many plates used are primarily for stabilization during healing and are not subjected to the high loads seen in major long bones. Customization can be beneficial for complex fractures or unusual anatomies.
Why Customization Matters in These Areas:
- Complex Anatomy: The craniofacial skeleton, hands, and feet have intricate three-dimensional structures. Standard plates often require significant intraoperative bending, which can weaken the plate and compromise the fit.
- Ästhetik: In facial reconstruction, achieving symmetry and restoring natural contours is critical for the patient’s quality of life. Custom plates provide superior cosmetic outcomes.
- Thin Soft Tissue Coverage: In areas like the scalp or face, bulky or poorly fitting plates can be palpable or visible, or even lead to skin breakdown. Low-profile, precisely contoured custom plates minimize these risks.
- Reduzierte Operationszeit: As mentioned, eliminating the need for manual plate bending significantly shortens operative duration, reducing anesthesia time and potential complications.
- Improved Stability: A perfect fit distributes stress more evenly and provides more secure fixation, promoting reliable healing.
Considerations for Suppliers and Distributors:
The shift towards custom implants impacts the traditional supply chain model. While a stock of standard plates remains necessary for emergency trauma, there’s a growing demand from hospitals and surgical centers for reliable partners who can facilitate the entire custom implant workflow:
- Image Acquisition & Segmentation: Protocols for obtaining high-quality CT/MRI scans and accurately converting them into 3D models.
- Design Collaboration: Platforms or services that allow surgeons to collaborate with engineers on the implant design.
- Manufacturing Expertise: Access to validated metal 3D printing processes (like SEBM or SLM) using certified medical-grade materials (like Ti-6Al-4V ELI). Companies like Met3dp, with their integrated approach covering advanced powder manufacturing and printing systems, are crucial here.
- Qualitätssicherung: Rigorous inspection and documentation processes meeting regulatory standards (e.g., ISO 13485).
- Lead Time Management: Efficient processes to deliver custom implants within clinically acceptable timeframes.
The applications for non-load-bearing custom 3D printed bone plates are diverse and expanding. They represent a significant advancement in personalized surgery, offering tangible benefits in complex anatomical regions where precision, fit, and aesthetics are paramount. For healthcare providers and their procurement partners, embracing this technology means partnering with knowledgeable manufacturers and suppliers capable of delivering these sophisticated, patient-specific solutions.
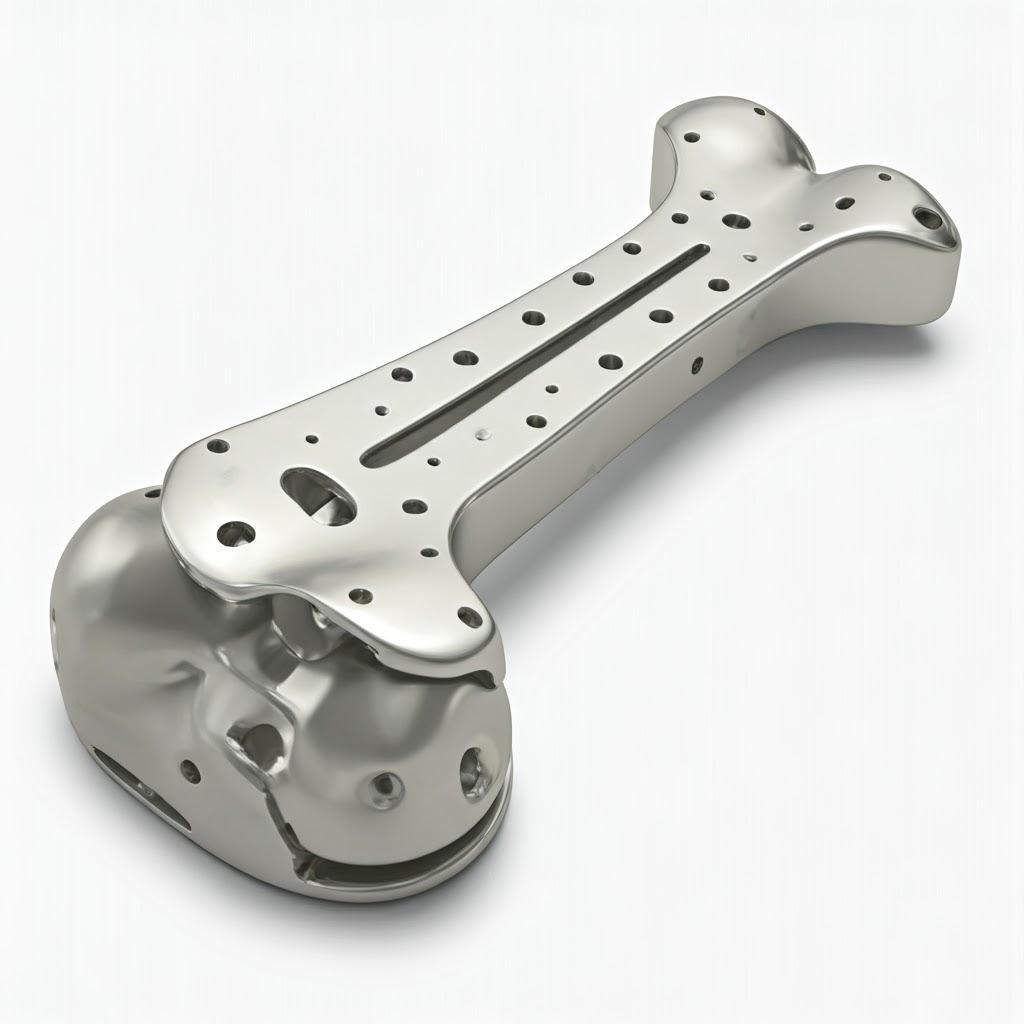
Why Use Metal 3D Printing for Custom Bone Plates? Key Advantages for Patients and Providers
The adoption of metal additive manufacturing (AM) for producing non-load-bearing bone plates, particularly patient-specific ones, isn’t just a novel approach; it offers a compelling suite of advantages over traditional manufacturing methods for patients, surgeons, hospitals, and medical device distributors alike. While conventional techniques like CNC machining or casting have served orthopedics for decades, they inherently limit design complexity and customization potential. Metal 3D printing overcomes these limitations, ushering in significant improvements in clinical outcomes, operational efficiency, and supply chain management.
1. Unparalleled Patient-Specific Anatomical Fit:
- The Core Benefit: This is the most significant advantage. Using data from a patient’s CT or MRI scan, a 3D model of the affected bone is created. The bone plate is then digitally designed to perfectly conform to the unique contours and geometry of that specific patient’s anatomy.
- Clinical Impact:
- Improved Stability: A plate that fits perfectly distributes stress more evenly across the bone-implant interface, leading to more stable fixation and reducing the risk of micromotion, which can impede healing.
- Reduced Malunion/Nonunion Risk: Precise anatomical reduction facilitated by a custom plate lowers the chances of the bone healing in an incorrect position (malunion) or failing to heal altogether (nonunion).
- Enhanced Functional Outcomes: In areas like the jaw or orbit, precise restoration of anatomy is crucial for function (e.g., proper bite, normal eye movement).
- Superior Aesthetics: In craniofacial reconstruction, a perfectly contoured plate restores natural appearance far better than a bent standard plate.
- Traditional Limitations: Standard plates come in pre-set sizes and shapes. Surgeons must often bend these plates extensively during surgery to approximate the patient’s anatomy. This bending process is time-consuming, relies heavily on surgeon experience, can weaken the plate, and may never achieve a truly perfect fit.
2. Design Freedom for Complex Geometries:
- Beyond Simple Shapes: AM builds parts layer by layer, allowing for the creation of highly complex shapes that are difficult or impossible to produce with traditional methods.
- Clinical Applications:
- Integrated Porous Structures: Plates can be designed with lattice or trabecular structures on the bone-contacting surface or throughout the plate body (where structurally appropriate). These pores mimic the structure of cancellous bone, encouraging osseointegration – the direct growth of bone into the implant – leading to better long-term stability and biological fixation.
- Optimized Shapes: Plates can be designed with variable thickness, internal channels, or unique contours to avoid sensitive anatomical structures, accommodate specific screw trajectories, or minimize overall implant volume and weight while maintaining necessary strength.
- Consolidated Components: Features that might require multiple parts in a traditional assembly could potentially be integrated into a single 3D printed component.
- Traditional Limitations: Machining is limited by tool access, making internal channels or complex porous networks extremely difficult. Casting has limitations in terms of achievable detail and porosity control.
3. Reduced Surgical Time and Complexity:
- Eliminating Intraoperative Bending: Because the plate arrives pre-contoured to the patient’s specific anatomy, the time-consuming and often challenging step of bending the plate during surgery is eliminated or significantly reduced.
- Clinical Impact:
- Shorter Anesthesia Duration: Reduced operating time translates to less time under anesthesia for the patient, lowering associated risks.
- Lower Blood Loss: Shorter procedures typically correlate with reduced intraoperative blood loss.
- Increased OR Efficiency: Faster turnaround times improve operating room utilization, benefiting hospital logistics and potentially reducing waiting lists.
- Reduced Surgeon Fatigue: Simplifying the procedure can lessen surgeon fatigue, potentially improving focus and performance.
- Planning Tools: The digital design process often involves surgical planning software, allowing surgeons to visualize the procedure, plan screw placements, and anticipate challenges before entering the operating room. Custom surgical guides can also be 3D printed alongside the plate to ensure accurate placement.
4. Material Efficiency and Reduced Waste:
- Additiv vs. Subtraktiv: AM builds parts by adding material only where needed. In contrast, traditional machining starts with a larger block of material and removes (subtracts) excess material to achieve the final shape.
- Auswirkungen:
- Less Material Consumption: Especially with expensive medical-grade alloys like Ti-6Al-4V ELI, reducing material waste translates to cost savings and better resource utilization. While unused powder in PBF processes can often be recycled, the overall buy-to-fly ratio (ratio of raw material weight to final part weight) is generally much better in AM compared to machining complex parts.
- Nachhaltigkeit: Less waste contributes to more environmentally sustainable manufacturing practices.
5. Enhanced Supply Chain and Inventory Management:
- Produktion auf Abruf: Custom plates are manufactured specifically for each patient, moving away from the need for hospitals and distributors to stock large inventories of standard plates in numerous sizes and configurations.
- Impact for Providers and Distributors:
- Geringere Lagerkosten: Lower capital tied up in stock, reduced storage space requirements, and less risk of implant expiration or obsolescence.
- Streamlined Logistics: Simplified ordering process focused on patient-specific needs rather than bulk standard parts.
- Potential for Point-of-Care: While still evolving, the long-term vision includes the possibility of hospitals having in-house or regional AM facilities for faster turnaround of custom implants, further revolutionizing the supply chain. Reliable Anbieter von 3D-Metalldruckdiensten like Met3dp are essential partners in realizing this potential, whether through centralized production or supporting localized facilities.
- Traditional Model: Requires significant forecasting, bulk purchasing, and complex inventory management by distributors and hospitals to ensure the availability of appropriate standard implant sizes.
6. Facilitation of Innovation:
- Rapid Prototyping: AM allows for rapid iteration of new implant designs. Engineers and surgeons can quickly test and refine novel concepts for non-load-bearing plates, accelerating the pace of innovation in orthopedic treatments.
- Novel Solutions: The design freedom enables entirely new approaches to fixation and reconstruction that were previously inconceivable.
Summary Table: AM vs. Traditional Manufacturing for Bone Plates
Merkmal | Additive Fertigung von Metall (3D-Druck) | Traditional Manufacturing (Machining/Casting) |
---|---|---|
Personalisierung | Patient-specific, high anatomical conformity | Standard sizes, requires manual bending |
Geometrische Komplexität | High (porous structures, internal channels, complex curves) | Limited by tooling/molding constraints |
Anatomical Fit | Excellent, pre-contoured | Approximate, relies on intraoperative bending |
Intraoperative Time | Reduced (no/less bending) | Increased (requires bending) |
Osseointegration | Potential for designed porous structures | Limited (surface treatments possible) |
Materialabfälle | Niedriger (additives Verfahren) | Higher (subtractive process) |
Inventory Model | On-demand, reduced stock | Requires large inventory of standard sizes |
Entwurf Iteration | Fast, facilitates rapid prototyping | Slower, more costly |
Vorlaufzeit (kundenspezifisch) | Days to weeks (process dependent) | N/A (standard parts readily available) |
Lead Time (Standard) | N/A (can produce standard if needed) | Immediate (if in stock) |
In Blätter exportieren
In conclusion, metal 3D printing, particularly using advanced systems and high-quality materials from providers like Met3dp, offers a cascade of benefits for the production of custom non-load-bearing bone plates. From the fundamental advantage of a perfect patient fit, leading to better clinical results, to operational efficiencies in the OR and optimized supply chains for wholesale distributors and hospitals, AM represents a superior technological approach for these demanding orthopedic applications.
Ti-6Al-4V ELI: The Gold Standard Material for 3D Printed Bone Plates
The success of any medical implant hinges critically on the material from which it is made. For 3D printed non-load-bearing bone plates, the overwhelming material of choice is Ti-6Al-4V ELI (Güte 23). This specific titanium alloy has earned its reputation as the gold standard due to an exceptional combination of properties that make it uniquely suited for long-term implantation in the human body and compatible with additive manufacturing processes. Understanding why Ti-6Al-4V ELI is preferred requires delving into its composition, mechanical properties, biocompatibility, and the specific requirements of metal powder for 3D printing.
Composition and the Significance of ‘ELI’:
Ti-6Al-4V, also known as Grade 5 titanium, is an alpha-beta titanium alloy. Its nominal composition is:
- Titanium (Ti): Base element
- Aluminum (Al): ~6% (Alpha phase stabilizer, increases strength)
- Vanadium (V): ~4% (Beta phase stabilizer, increases strength)
The “ELI” designation stands for Extra Low Interstitials. This means that the maximum allowable limits for interstitial elements – primarily oxygen (O), nitrogen (N), carbon (C), and iron (Fe) – are significantly lower than in standard Grade 5 titanium.
- Standard Ti-6Al-4V (Grade 5): Typically allows higher levels of Oxygen (e.g., ≤ 0.20%).
- Ti-6Al-4V ELI (Grade 23): Mandates lower levels (e.g., Oxygen ≤ 0.13%).
Why are low interstitials crucial for medical implants?
Interstitial elements, even in small amounts, can significantly impact the alloy’s mechanical properties. Higher levels generally increase strength but reduce ductility (the ability to deform without fracturing) and fracture toughness (resistance to crack propagation). For a medical implant like a bone plate, especially one with complex geometry created via 3D printing, ductility and fracture toughness are paramount for safety and long-term performance. Lowering the interstitial content makes Ti-6Al-4V ELI more forgiving, less brittle, and more resistant to fatigue failure compared to standard Grade 5, making it the preferred choice for critical applications like surgical implants.
Key Properties of Ti-6Al-4V ELI for Bone Plates:
- Ausgezeichnete Biokompatibilität: This is non-negotiable for any implant material.
- Inertness: Titanium naturally forms a stable, thin, and adherent oxide layer (TiO2) on its surface almost instantly when exposed to air or bodily fluids. This layer is highly resistant to corrosion in the body’s saline environment and prevents the leaching of metal ions into surrounding tissues.
- Low Toxicity: Titanium and its common alloying elements (Aluminum and Vanadium in these quantities) have demonstrated excellent biocompatibility with minimal adverse tissue reactions or systemic toxicity.
- Osseointegration: While inherently bioinert, the surface of titanium readily allows for bone cells (osteoblasts) to attach and proliferate, leading to direct bone apposition onto the implant surface – a process called osseointegration. This is crucial for the long-term stability of orthopedic implants. 3D printing allows for the intentional design of porous surfaces that further enhance this biological fixation.
- Hohes Verhältnis von Festigkeit zu Gewicht:
- Titanium alloys are significantly lighter than stainless steels or Cobalt-Chrome alloys (other common implant materials) but offer comparable or superior strength.
- This results in strong, stable fixation without adding excessive weight or bulk, which is particularly important in craniofacial applications where minimizing implant profile is often desired.
- Ausgezeichnete Korrosionsbeständigkeit:
- The stable TiO2 passive layer provides exceptional resistance to corrosion and degradation in the physiological environment, ensuring the implant’s structural integrity over its intended lifespan.
- Appropriate Mechanical Properties:
- Elastizitätsmodul: Ti-6Al-4V ELI has a modulus of elasticity (stiffness) of around 110-115 GPa. While still stiffer than natural bone (typically 10-30 GPa), it is significantly lower than stainless steel (~200 GPa) or CoCr alloys (~240 GPa). This lower modulus can reduce “stress shielding” – a phenomenon where a very stiff implant carries too much load, shielding the adjacent bone from normal physiological stresses, potentially leading to bone resorption (weakening) over time. While less critical for non-load-bearing plates than for hip stems, a closer match to bone’s modulus is generally favorable.
- Ermüdungsfestigkeit: Implants are subjected to cyclic loading, even in non-load-bearing areas (e.g., from muscle pulls, minor impacts). Ti-6Al-4V ELI exhibits excellent fatigue strength, ensuring durability under physiological conditions. The ELI grade’s enhanced fracture toughness further contributes to fatigue resistance.
- Zugfestigkeit: Possesses high tensile strength (typically >830 MPa in the annealed state) to withstand functional loads without permanent deformation.
- MRI and CT Compatibility:
- Titanium is non-ferromagnetic, making it safe for patients undergoing MRI scans. While it does produce some artifacts (distortions) in MRI and CT images, these are generally less severe than those caused by stainless steel or CoCr alloys, allowing for better post-operative imaging of surrounding tissues.
Powder Requirements for Additive Manufacturing:
Producing high-quality Ti-6Al-4V ELI bone plates via AM requires starting with metal powder that meets stringent specifications. The characteristics of the powder directly influence the printing process stability and the final part’s density, microstructure, and mechanical properties. Key powder attributes include:
- Sphärizität: Powder particles should ideally be highly spherical. This promotes good powder flowability (the ability of the powder to spread evenly across the build platform) and ensures uniform packing density, leading to consistent melting and fully dense final parts.
- Partikelgrößenverteilung (PSD): The range and distribution of particle sizes must be carefully controlled and optimized for the specific AM process (e.g., SLM or SEBM). A suitable PSD ensures good powder bed density and efficient melting. Typical ranges might be 15-53 µm or 45-105 µm depending on the machine and application.
- Fließfähigkeit: As mentioned, good flowability is essential for uniformly recoating the powder bed layer after layer. Poor flow can lead to defects like porosity.
- Chemische Reinheit: The powder must strictly adhere to the Ti-6Al-4V ELI chemical composition standards (e.g., ASTM F136), with minimal contaminants. Oxygen pickup during powder production and handling must be tightly controlled.
- Absence of Satellites and Internal Porosity: High-quality powder production aims to minimize smaller particles attached to larger ones (satellites) and internal gas pores within the powder particles, both of which can negatively impact processing and final part quality.
Met3dp’s Expertise in Powder Production:
Ensuring a consistent supply of high-quality Ti-6Al-4V ELI powder meeting these demanding criteria is crucial for medical device manufacturers and wholesale suppliers. This is where specialized powder producers like Met3dp play a vital role.
- Fortgeschrittene Zerstäubungstechniken: Met3dp employs industry-leading gas atomization (GA) and Plasma Rotating Electrode Process (PREP) technologies.
- Gaszerstäubung: Uses high-pressure inert gas jets to break up a stream of molten Ti-6Al-4V ELI into fine droplets that solidify into spherical powder. Met3dp utilizes unique nozzle and gas flow designs to optimize sphericity and flowability while minimizing contamination.
- PREP: Rotates a consumable electrode of the desired alloy at high speed in an inert atmosphere. A plasma torch melts the tip of the electrode, and centrifugal force ejects molten droplets that solidify in flight, typically yielding highly spherical powders with very few satellites or internal porosity – ideal for critical applications.
- Qualitätskontrolle: Rigorous quality control measures, including chemical analysis, PSD measurement, morphology assessment (e.g., via Scanning Electron Microscopy), and flowability testing, ensure that every batch of Met3dp’s Ti-6Al-4V ELI powder meets the specifications required for demanding metal 3D printing applications, especially in the medical field.
Comparison Table: Ti-6Al-4V ELI vs. Other Implant Materials
Eigentum | Ti-6Al-4V ELI (Güte 23) | 316L-Edelstahl | CoCr Alloy (ASTM F75) | PEEK (Polymer) |
---|---|---|---|---|
Primäre Verwendung | Orthopedics, Dental | Orthopedics (Trauma) | Orthopedics (Joints) | Spinal, Craniofacial |
Biokompatibilität | Ausgezeichnet | Gut | Gut | Ausgezeichnet |
Dichte (g/cm³) | ~4.43 | ~8.0 | ~8.3-9.2 | ~1.3 |
Elastizitätsmodul (GPa) | ~114 | ~193 | ~210-240 | ~3.6 |
Zugfestigkeit (MPa) | >830 | >515 | >655 | ~100 |
Korrosionsbeständigkeit | Ausgezeichnet | Good (Pitting risk) | Sehr gut | Ausgezeichnet |
MRI-Kompatibilität | Good (Non-ferromagnetic) | Poor (Ferromagnetic) | Fair (Paramagnetic) | Ausgezeichnet |
Osseointegration | Good (Enhanced by pores) | Schlecht | Messe | Poor (Bioinert) |
3D Printability | Ausgezeichnet | Gut | Gut | Possible (FDM/SLS) |
In Blätter exportieren
In summary, Ti-6Al-4V ELI’s unique blend of biocompatibility, mechanical strength, ductility, fracture toughness, corrosion resistance, and suitability for advanced manufacturing processes like SEBM and SLM firmly establishes it as the material of choice for 3D printed non-load-bearing bone plates. The stringent control over interstitial elements in the ELI grade provides an added margin of safety crucial for medical implants. Access to high-quality, contamination-free powder with optimal characteristics, such as that produced by Met3dp using advanced atomization techniques, is fundamental to realizing the full potential of this material in creating next-generation, patient-specific orthopedic solutions. Sources and related content
Design Considerations for Additively Manufactured Bone Plates: Optimizing for Fit and Function
The remarkable advantage of metal additive manufacturing (AM) lies in its ability to create patient-specific bone plates with unparalleled anatomical conformity and potentially enhanced biological function. However, unlocking this potential requires more than just access to a 3D printer; it demands a thoughtful and sophisticated approach to design, specifically Design for Additive Manufacturing (DfAM). Designing a custom 3D printed bone plate is an iterative, collaborative process that integrates medical imaging data, biomechanical principles, surgical requirements, and the specific capabilities and constraints of the chosen AM technology (like SLM or SEBM) and material (Ti-6Al-4V ELI). For medical device engineers, surgeons, and the procurement teams sourcing these custom solutions, understanding these design considerations is paramount.
1. High-Fidelity Input Data: The Foundation
The entire process begins with acquiring high-quality medical imaging data, typically Computed Tomography (CT) scans of the patient’s relevant anatomy.
- Scan Quality: The resolution, slice thickness, and overall quality of the CT scan directly impact the accuracy of the resulting 3D model and, consequently, the fit of the final implant. Standardized protocols for implant planning scans are essential. MRI scans may also be used, particularly for visualizing soft tissues, but CT generally provides better bone definition.
- Image Segmentation: Specialized software is used to convert the 2D scan data (DICOM format) into a 3D anatomical model (often in STL or similar formats). This crucial step, called segmentation, involves identifying and isolating the specific bone(s) of interest from surrounding tissues. Accuracy here is critical and often requires skilled technicians or AI-assisted tools. Errors in segmentation will directly translate into inaccuracies in the implant design.
- Data Integrity: Ensuring patient data privacy and security throughout this digital workflow is non-negotiable, adhering to regulations like HIPAA or GDPR.
2. Anatomical Conformity and Plate Contouring:
- Virtual Fitting: The core design task involves creating the plate geometry to perfectly match the 3D model of the patient’s bone surface. CAD (Computer-Aided Design) software allows designers to virtually “drape” or sculpt the plate onto the bone model.
- Plate Boundaries and Thickness: The extent of the plate (how far it reaches) and its thickness profile are critical. Thickness can be varied along the plate’s length – potentially thicker over the fracture site or defect and thinner at the edges to minimize palpability under thin skin (especially in craniofacial areas). DfAM allows for smooth transitions and organic shapes that minimize stress concentrations.
- Avoiding Critical Structures: The design must carefully consider nearby nerves, blood vessels, tooth roots, or other sensitive anatomical structures, ensuring the plate and screws avoid impinging on them.
3. Screw Placement and Trajectory:
- Optimal Fixation: Screw holes must be positioned strategically to achieve stable fixation in areas of good quality bone, avoiding fracture lines or areas of bone loss.
- Screw Angulation: AM allows for screw holes to be designed with specific angles, enabling surgeons to direct screws for optimal purchase (e.g., bicortical fixation) or to avoid underlying structures. Locking screw technology, where the screw head threads into the plate hole, can also be incorporated for enhanced stability, creating a fixed-angle construct. The design must ensure sufficient material around the screw hole to support locking mechanisms.
- Surgical Access: Consideration must be given to how the surgeon will access the screw holes during the operation. Hole placement and angulation must allow for feasible drill and screwdriver access.
4. Incorporating Porous Structures and Lattice Designs:
This is a unique capability offered by AM, aiming to enhance osseointegration.
- Zweck: By designing controlled porosity into the bone-contacting surface or even parts of the plate’s body, the implant can encourage bone cells to grow into its structure, leading to stronger biological fixation over time. This can be particularly beneficial for long-term stability and load transfer.
- Design Parameters:
- Unit Cell Type: Various repeating geometric shapes (e.g., cubic, diamond, gyroid) can be used to create the lattice. The choice affects mechanical properties, permeability, and printability.
- Porosity Percentage: The overall void space within the lattice (e.g., 50-80%). Higher porosity encourages ingrowth but reduces mechanical strength.
- Strut/Wall Thickness: The diameter of the individual beams or walls forming the lattice. Must be sufficient for printability and structural integrity but thin enough to allow cell migration and vascularization. Minimum printable feature size of the AM system is a key constraint.
- Standort: Porosity is typically applied to the bone-facing surface or in specific regions where biological fixation is prioritized and mechanical stress is lower. Fully porous plates are generally not feasible due to strength requirements.
- Topologie-Optimierung: Advanced software tools can automatically optimize the distribution of material within the plate design based on expected loads, minimizing weight and volume while maintaining necessary strength and stiffness. This can lead to highly organic-looking, efficient structures.
5. Design for Manufacturability (DfAM Principles):
Designing for AM is different from designing for machining. Key considerations include:
- Unterstützende Strukturen: Most PBF processes require support structures to anchor the part to the build plate, prevent warping due to thermal stress, and support overhanging features during the build. These supports must be removed in post-processing. Good design practice aims to:
- Überhänge minimieren: Orienting the part on the build plate to reduce the need for supports. Self-supporting angles (typically >45 degrees from the horizontal for titanium) should be utilized where possible.
- Design Accessible Supports: Ensuring supports can be easily accessed and removed without damaging the implant surface. Support contact points should ideally be on non-critical surfaces.
- Wanddicke und Größe der Merkmale: There are minimum printable wall thicknesses and feature sizes dictated by the AM process (laser/electron beam spot size, powder particle size). Designs must adhere to these limits (e.g., typically >0.3-0.5 mm for walls). Sharp internal corners should be avoided (use fillets) to reduce stress concentrations.
- Reststress-Management: Part orientation and geometry can influence the buildup of internal stresses during the layer-by-layer heating and cooling cycles. Design choices, alongside optimized process parameters, can help mitigate warping or cracking.
- Entfernung von Puder: For parts with internal channels or complex lattice structures, ensuring that unfused powder can be completely removed after printing is critical. Trapped powder is unacceptable in a medical implant. Design considerations include incorporating drainage holes or ensuring channels are large enough for effective cleaning.
6. Collaboration and Workflow:
- Surgeon-Engineer Partnership: Effective custom implant design requires close collaboration between the surgeon (who understands the clinical need and anatomy) and the biomedical engineer (who understands DfAM principles and material science). Iterative design reviews are common.
- Software-Tools: A range of software is used: Medical image processing software, CAD software, simulation software (FEA – Finite Element Analysis) to predict mechanical performance under load, and AM build preparation software.
- Regulatory Considerations: Designs must comply with relevant medical device regulations (e.g., FDA guidelines, MDR in Europe). Design history files, risk assessments, and validation documentation are essential components required by medical device manufacturers and expected by their wholesale distributors and hospital customers.
Engaging with an experienced 3D-Druck-Dienstleister für Metall like Met3dp, who possesses expertise not only in printing but also in design optimization for medical applications using materials like Ti-6Al-4V ELI, can significantly streamline this complex process and ensure the final implant meets the demanding requirements for fit, function, and safety. Their understanding of the capabilities of their specific AM systems (like high-precision SEBM) informs the design process from the outset.
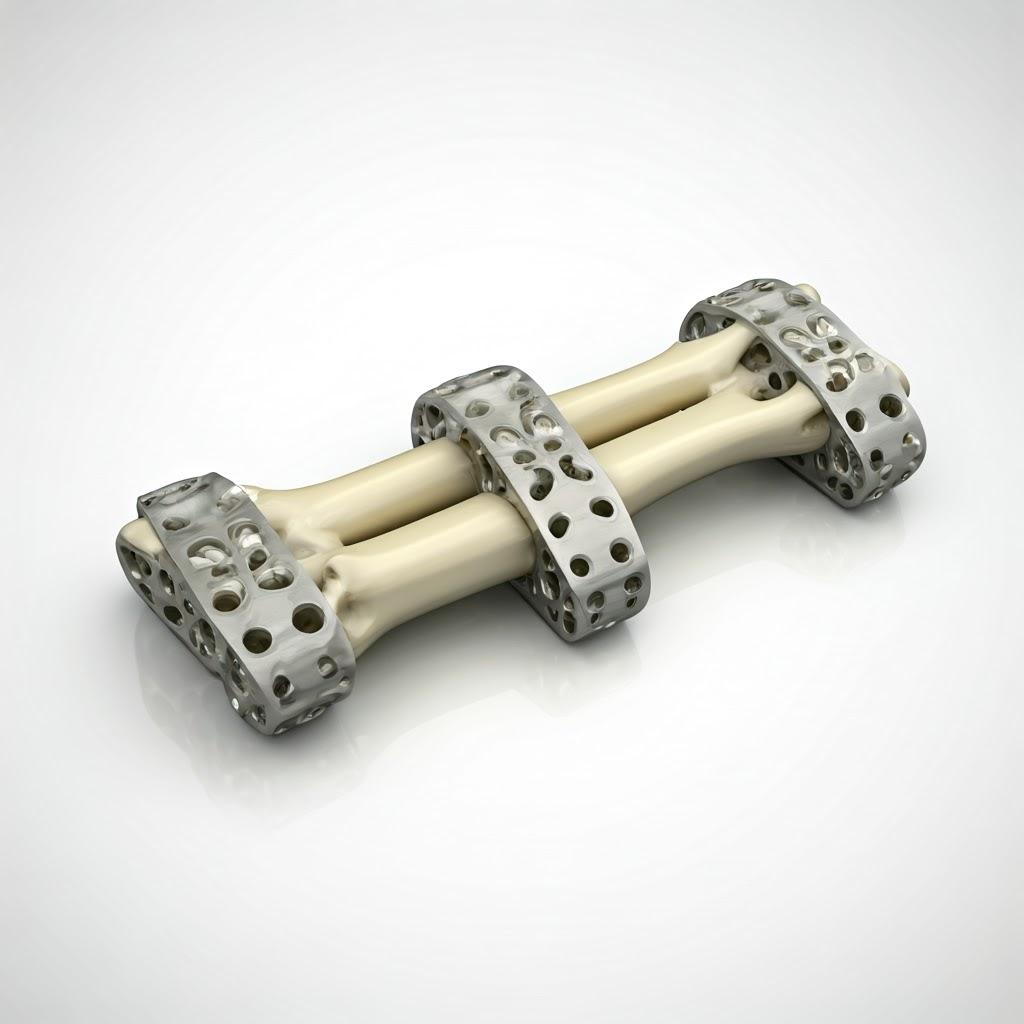
Erreichen von Präzision: Toleranz, Oberflächengüte und Maßgenauigkeit bei 3D-gedruckten Implantaten
While the design freedom of metal additive manufacturing is a major draw, the resulting implants must also meet stringent requirements for precision. Dimensional accuracy, appropriate tolerances, and controlled surface finish are critical for the proper function, safety, and biocompatibility of 3D printed non-load-bearing bone plates. Engineers designing these components, manufacturers producing them, and procurement specialists sourcing them need a clear understanding of what precision levels are achievable with current AM technologies like SLM and SEBM, the factors influencing them, and the role of post-processing.
Maßgenauigkeit und Toleranzen:
Dimensional accuracy refers to how closely the final manufactured part conforms to the nominal dimensions specified in the digital CAD model. Tolerance defines the permissible range of variation for a given dimension.
- Achievable Tolerances (As-Built): For Ti-6Al-4V ELI parts produced using well-controlled PBF processes (SLM/SEBM), typical as-built dimensional tolerances are often in the range of:
- ± 0.1 mm to ± 0.3 mm for smaller features (< 50 mm)
- ± 0.2% to ± 0.5% of the nominal dimension for larger features. These values are general guidelines and can vary significantly based on part geometry, size, build orientation, specific machine calibration, and process parameters used. Companies like Met3dp, through rigorous process control and advanced machine technology like SEBM, strive for high levels of accuracy and repeatability.
- Faktoren, die die Genauigkeit beeinflussen:
- Thermische Effekte: The rapid melting and solidification inherent in PBF processes lead to thermal expansion and contraction, causing internal stresses that can result in slight warping or shrinkage. Careful thermal management during the build and appropriate heat treatment afterwards are crucial.
- Orientierung aufbauen: The orientation of the part on the build platform affects thermal gradients, support structure requirements, and the “stair-stepping” effect on curved or angled surfaces, all influencing final accuracy.
- Laser/Electron Beam Spot Size: The diameter of the energy beam limits the minimum feature size and influences the precision of fine details.
- Eigenschaften des Pulvers: Variations in powder particle size distribution or morphology can affect melt pool stability and dimensional consistency.
- Kalibrierung der Maschine: Regular and precise calibration of the AM system (scanners, energy source, Z-axis movement) is essential.
- Meeting Critical Tolerances: While as-built tolerances are often sufficient for the overall anatomical conformity of a bone plate, certain features may require tighter control. For example:
- Screw Holes: Diameter and locking feature geometry might require tolerances tighter than achievable in the as-built state.
- Mating Surfaces: Interfaces designed to connect with other components or surgical instruments may need higher precision. In such cases, secondary machining operations are typically employed after printing.
Oberflächengüte (Rauhigkeit):
Surface finish, typically quantified by average roughness (Ra), is another critical characteristic of 3D printed implants.
- As-Built Surface Roughness: PBF processes inherently produce parts with a noticeable surface roughness due to the layer-by-layer nature and the partially melted powder particles adhering to the surface. Typical as-built Ra values for Ti-6Al-4V ELI can range from:
- 5 µm to 20 µm (micrometers) or even higher, depending on the surface orientation (upward-facing, downward-facing, vertical walls) and process parameters. Downward-facing surfaces requiring supports often exhibit higher roughness after support removal.
- Einfluss der Oberflächenbeschaffenheit:
- Biokompatibilität: While titanium’s inherent biocompatibility is excellent, excessive roughness can potentially increase surface area for ion release (though typically minimal with stable TiO2 layer) and may influence initial cell interactions. Very rough surfaces might also potentially harbor bacteria if not properly cleaned and sterilized, although this is debated.
- Müdigkeit Leben: Surface roughness can act as initiation sites for fatigue cracks. Smoother surfaces generally lead to better fatigue performance, which is important even for non-load-bearing plates subjected to minor cyclic loads.
- Friction and Wear: While less critical for static bone plates than for articulating joint implants, surface finish can influence friction if the plate interacts with soft tissues or instrumentation.
- Verbesserung der Oberflächengüte: As-built surfaces are rarely acceptable for final implantation without modification. Various post-processing techniques are used to reduce roughness:
- Abrasives Strahlen (Perlstrahlen): Common for achieving a uniform matte finish and removing loosely adhering particles (Ra typically 3-6 µm).
- Mass Finishing (Tumbling, Vibratory Finishing): Using abrasive media in a rotating or vibrating drum to smooth surfaces and round edges (Ra can reach 1-3 µm).
- Elektropolieren: An electrochemical process that removes a microscopic layer of material, resulting in a very smooth, clean, and corrosion-resistant surface (Ra often < 0.5 µm).
- Manuelles Polieren: Labor-intensive but can achieve mirror-like finishes on specific areas if required.
Quality Control and Validation:
Ensuring that each custom 3D printed bone plate meets the specified dimensional and surface finish requirements is crucial for patient safety and regulatory compliance. This involves:
- Prozess-Validierung: Medical device manufacturers must validate their entire AM process (including design, printing, post-processing, cleaning, sterilization) to demonstrate consistent output according to specifications (e.g., following ISO 13485 quality management standards).
- Prüfung der Abmessungen: Using tools like Coordinate Measuring Machines (CMMs), 3D scanners (structured light or laser), and traditional calipers/micrometers to verify critical dimensions against the CAD model and drawings.
- Messung der Oberflächenrauhigkeit: Employing profilometers to quantify surface roughness (Ra, Rz, etc.) on critical surfaces.
- Zerstörungsfreie Prüfung (NDT): Techniques like X-ray or CT scanning can be used to inspect internal features (like lattice structures) and detect potential defects (like porosity or trapped powder) without damaging the part.
- Rückverfolgbarkeit: Maintaining complete traceability from the initial powder batch through design, printing, post-processing, and final inspection is essential for medical device quality systems. This is a key requirement for suppliers serving the medical wholesale market.
Summary Table: Precision Aspects
Parameter | Typical As-Built (Ti-6Al-4V ELI PBF) | Factors Influencing | Post-Processing Impact | Importance for Implants |
---|---|---|---|---|
Abmessungstoleranz | ±0.1-0.3mm / ±0.2-0.5% | Thermal stress, orientation, machine | Machining for tight tolerances | Critical for fit, screw function, mating interfaces |
Oberflächenrauhigkeit (Ra) | 5-20 µm+ | Orientation, supports, parameters | Blasting, tumbling, polishing | Biocompatibility, fatigue life, cell interaction, cleaning |
Merkmal Auflösung | ~0.1-0.3 mm | Beam spot size, powder size | Machining for fine details | Limits minimum strut size in lattices, sharp corners |
Innere Porosität | <0.1% (with optimization & HIP) | Process parameters, powder quality | HIP reduces/eliminates porosity | Critical for mechanical integrity, fatigue resistance |
In Blätter exportieren
In essence, while metal 3D printing offers incredible geometric freedom, achieving the necessary precision for medical implants like bone plates requires careful DfAM, tightly controlled printing processes (as offered by manufacturers utilizing advanced equipment like Met3dp’s SEBM systems), and meticulous, validated post-processing steps. Understanding the interplay between design, manufacturing, and finishing is key to producing safe, effective, and reliable patient-specific orthopedic solutions that meet both clinical needs and regulatory expectations.
Essential Post-Processing Steps for Titanium Bone Plates: Ensuring Safety and Performance
Creating a dimensionally accurate titanium bone plate with complex features via additive manufacturing is only part of the journey. The “as-built” part, fresh off the printer, is not yet suitable for implantation. A series of crucial post-processing steps are required to transform the raw printed component into a safe, biocompatible, and high-performance medical device. These steps address residual stresses, remove support structures, refine surface finish, ensure cleanliness, and verify quality. Medical device manufacturers, their suppliers, and hospital procurement teams must ensure these processes are validated and meticulously controlled according to medical device regulations (e.g., ISO 13485, FDA QSR).
1. Stressabbau / Wärmebehandlung:
- Zweck: The rapid heating and cooling cycles during PBF printing induce significant residual stresses within the Ti-6Al-4V ELI part. These stresses can cause distortion (warping) upon removal from the build plate or, worse, lead to premature failure in service. Heat treatment is essential to relieve these stresses and homogenize the material’s microstructure.
- Prozess:
- Stressabbau: Typically performed while the part is still attached to the build plate (or immediately after removal) in a vacuum or inert atmosphere furnace. Temperatures are usually in the range of 650-800°C, followed by controlled cooling. This reduces the internal stresses without significantly altering the microstructure obtained during printing.
- Heiß-Isostatisches Pressen (HIP): This is a highly recommended, often mandatory, step for critical implants. Parts are subjected to high temperature (e.g., 900-950°C for Ti-6Al-4V) and high inert gas pressure (e.g., 100-200 MPa) simultaneously. HIP effectively closes internal microporosity that might remain after printing, leading to a fully dense part (~99.9%+) with improved fatigue life and mechanical properties. It also achieves stress relief and some microstructural homogenization.
- Annealing/Solution Treatment & Aging: Further heat treatments may be applied after HIP or stress relief to optimize specific mechanical properties (e.g., strength, ductility) by modifying the alpha-beta phase microstructure, depending on the design requirements.
- Wichtigkeit: Failure to properly relieve stress can compromise dimensional stability and mechanical integrity. HIP significantly enhances fatigue performance, a critical safety factor.
2. Entfernen des Teils von der Bauplatte:
- Methode: The printed bone plate(s) are typically fused to a metal build plate during the AM process. They need to be carefully separated. Common methods include:
- Draht-Elektroerosion (Wire EDM): Precise and introduces minimal mechanical stress.
- Bandsägen: Faster but less precise and can induce more stress if not done carefully.
- Bearbeitungen: Milling the interface layer.
3. Entfernung der Stützstruktur:
- Zweck: Supports are necessary during printing but must be completely removed afterwards.
- Methoden:
- Manuelle Entfernung: Breaking or cutting away supports using hand tools (pliers, cutters). Labor-intensive and requires skill to avoid damaging the part surface.
- Spanende Bearbeitung (CNC): Milling or grinding support structures, especially in accessible areas.
- Drahterodieren: Kann für komplizierte oder schwer zugängliche Halterungen verwendet werden.
- Herausforderungen: Support removal can be difficult, especially for complex internal geometries or lattice structures. DfAM plays a crucial role in designing supports that are both effective and easier to remove. Incomplete support removal is unacceptable. Residual support “nubs” often require further blending or smoothing.
4. Surface Finishing:
- Zweck: To improve surface quality by reducing roughness, removing contaminants, and preparing the surface for optimal biocompatibility and performance.
- Common Techniques (often used in sequence):
- Strahlen (Perlen-/Kornstrahlen): Using compressed air to propel abrasive media (e.g., ceramic beads, aluminum oxide) onto the surface. Removes residual powder, smooths surfaces slightly, creates a uniform matte finish, and can remove minor support witness marks. Choice of media is critical to avoid contamination.
- Mass Finishing (Tumbling, Vibratory Finishing): Parts are placed in a tub with abrasive media and liquid compound. The tub rotates or vibrates, causing the media to rub against the parts, smoothing surfaces and deburring edges. Effective for batches of parts but less controlled for specific surface features.
- Manual Deburring and Blending: Using hand tools or power tools with abrasive tips to remove burrs and blend surfaces, particularly where supports were removed.
- Elektropolieren: An electrochemical process that preferentially removes peaks on the surface, resulting in a very smooth (low Ra), clean, and often brighter finish. It can also enhance corrosion resistance by thickening the passive oxide layer.
- Anodizing (Type II): An electrolytic process that grows a controlled oxide layer on the titanium surface. Often used to color-code implants for identification (different thicknesses produce different interference colors) but can also slightly improve wear resistance and potentially influence biocompatibility.
- Passivierung: A chemical treatment (typically using nitric or citric acid) to remove any free iron or other contaminants from the surface and ensure the formation of a robust, chromium-oxide-rich passive layer (more common for stainless steel, but sometimes applied to titanium).
5. Precision Machining (If Required):
- Zweck: To achieve tighter tolerances or specific surface finishes on critical features that cannot be met by the as-built AM process or subsequent finishing steps.
- Anwendungen: Refining screw hole diameters and threads (especially for locking screws), creating highly flat mating surfaces, machining specific functional features.
- Erwägungen: Requires careful fixture design to hold the complex AM part. Machining fluids must be biocompatible or completely removed.
6. Cleaning and Inspection:
- Zweck: This is an absolutely critical step to remove all contaminants from the manufacturing process, including residual powder, machining fluids, abrasive media, polishing compounds, and fingerprints. Implant cleanliness is paramount for biocompatibility and preventing adverse patient reactions or infection.
- Prozess: Typically involves a multi-stage process:
- Initial rinses: Removing gross contamination.
- Ultrasonic Cleaning: Using high-frequency sound waves in specialized cleaning solutions (detergents, solvents) to dislodge particles from intricate features and porous structures. Multiple baths with different solutions may be used.
- Final Rinses: Using purified or deionized water to remove cleaning agents.
- Drying: Using filtered hot air or vacuum ovens.
- Validierung: Cleaning processes must be rigorously validated to demonstrate their effectiveness in removing potential contaminants to acceptable levels, often verified through chemical analysis or cytotoxicity testing.
- Inspektion: Visual inspection (often under magnification), dimensional checks (CMM, scanning), surface roughness measurement, and potentially NDT (X-ray/CT) are performed to ensure the part meets all specifications before release.
7. Packaging and Sterilization:
- Verpackung: Cleaned and inspected implants are packaged in cleanroom environments, often double-bagged, with appropriate labeling providing full traceability (part number, batch number, material, patient ID if applicable).
- Sterilization: Packaged implants are terminally sterilized before surgical use. Common methods for titanium implants include:
- Autoklavieren (Dampfsterilisation): Most common method.
- Gamma Irradiation: Effective but can sometimes cause slight discoloration.
- Ethylene Oxide (EtO) Gas: Less common for metals due to potential residues. The chosen sterilization method must be validated to ensure it achieves the required Sterility Assurance Level (SAL) without adversely affecting the implant’s properties.
The Importance of Integrated Expertise:
Successfully navigating these intricate post-processing steps requires significant expertise and specialized equipment. Working with a vertically integrated partner or a highly qualified supplier network is crucial. Companies like Met3dp, offering solutions that span from powder production to advanced printing systems and application support, understand the entire value chain and the critical interplay between printing and post-processing required to deliver high-quality, implantable medical devices. Their focus on process control and material quality provides a strong foundation for subsequent finishing operations. Procurement teams evaluating potential suppliers must scrutinize their post-processing capabilities and quality management systems just as rigorously as their printing technology.
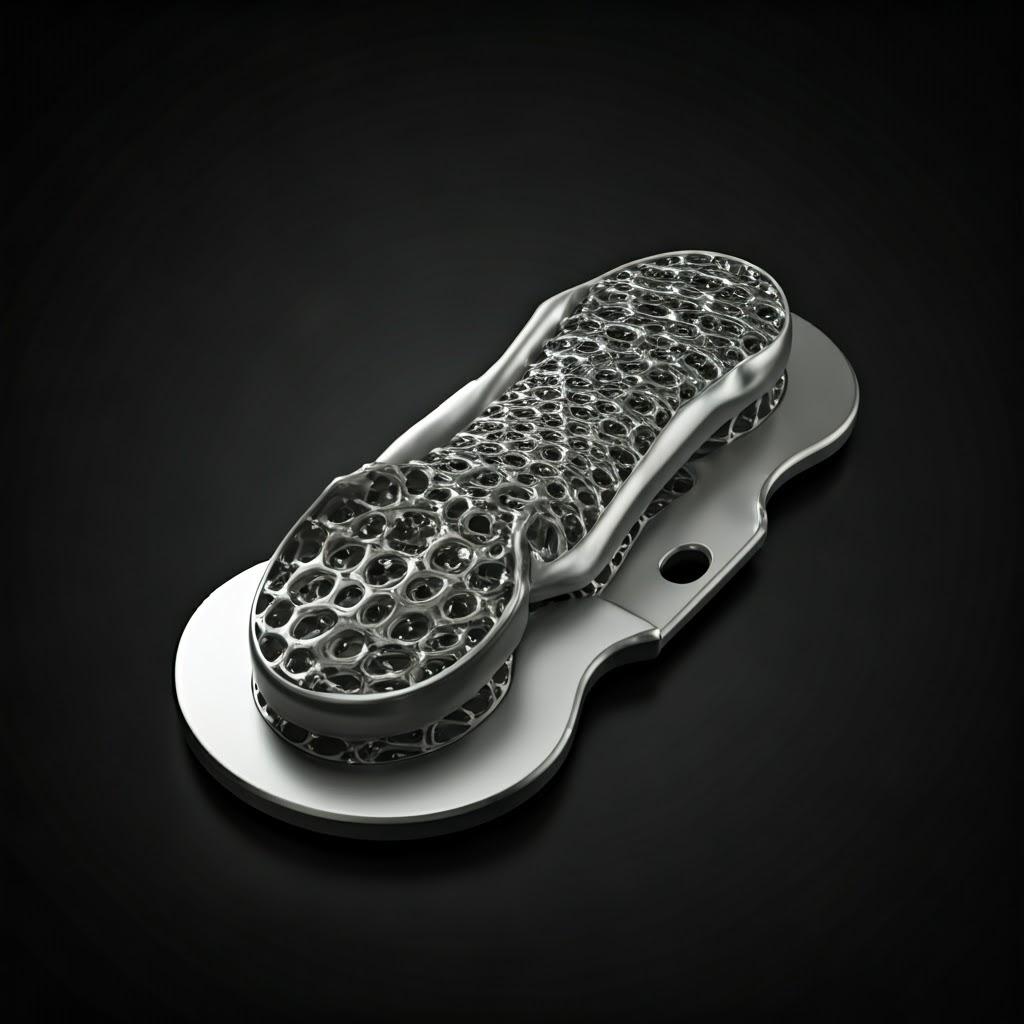
Navigating Challenges in 3D Printing Bone Plates: Solutions and Best Practices for Suppliers
While metal additive manufacturing offers transformative potential for custom bone plates, the journey from digital design to sterile implant is not without its challenges. Successfully implementing this technology requires anticipating potential issues and establishing robust processes and best practices to mitigate them. For medical device manufacturers, wholesale suppliers, and their production partners, understanding and addressing these challenges is key to ensuring consistent quality, regulatory compliance, and ultimately, patient safety.
1. Residual Stress, Distortion, and Cracking:
- Herausforderung: The rapid, localized heating and cooling inherent in PBF processes generate significant thermal gradients and resultant residual stresses within the Ti-6Al-4V ELI part. These stresses can cause parts to warp or distort, especially after removal from the build plate, or even lead to cracking during the build or subsequent handling.
- Lösungen und bewährte Praktiken:
- Optimierte Build-Strategie: Careful selection of build orientation, strategic placement of support structures (acting as heat sinks and anchors), and optimized scan strategies (e.g., island scanning, specific hatch patterns) can minimize stress accumulation.
- Optimierung der Prozessparameter: Fine-tuning parameters like laser/beam power, scan speed, layer thickness, and chamber heating (especially relevant in SEBM) is crucial for stable melting and reduced thermal shock.
- Obligatorische Wärmebehandlung: Implementing validated stress relief cycles (often on the build plate) and/or HIP is non-negotiable for mitigating residual stress and preventing distortion and cracking in titanium parts.
- DfAM: Designing parts with features that minimize stress concentration (e.g., fillets instead of sharp corners) and avoiding large, abrupt changes in cross-section can help.
2. Porosity and Lack of Fusion Defects:
- Herausforderung: Incomplete melting of powder particles or instabilities in the melt pool can lead to voids (porosity) within the final part. These defects can significantly compromise mechanical properties, particularly fatigue strength, acting as crack initiation sites. Porosity can arise from incorrect process parameters, poor powder quality, or gas entrapment.
- Lösungen und bewährte Praktiken:
- Rigorous Parameter Development: Extensive testing (developing parameter “coupons”) to establish optimal settings for density (>99.5% typically required before HIP) for the specific machine, material batch, and geometry type.
- Hochwertiges Pulver: Using powder with controlled sphericity, PSD, flowability, and low internal gas content is critical. Sourcing powder from reputable suppliers like Met3dp, who utilize advanced atomization (GA, PREP) and strict quality control, is essential. Regular powder testing and careful handling/recycling protocols are needed to prevent degradation.
- Prozessüberwachung: Advanced AM systems may incorporate in-situ monitoring tools (e.g., melt pool monitoring) to detect anomalies during the build, allowing for potential intervention or part rejection.
- Heiß-Isostatisches Pressen (HIP): As mentioned, HIP is highly effective at closing internal gas porosity, significantly improving part integrity.
- NDT-Inspektion: Using X-ray or micro-CT scanning to detect and quantify internal porosity in finished parts, especially for critical applications.
3. Support Structure Removal Difficulties:
- Herausforderung: Supports, while necessary, can be difficult and time-consuming to remove, especially from complex internal channels or delicate lattice structures. Incomplete removal or damage to the part surface during removal is a risk.
- Lösungen und bewährte Praktiken:
- DfAM-Optimierung: Designing parts and selecting build orientations to minimize the need for supports (using self-supporting angles) and ensuring necessary supports are accessible and designed for easier detachment (e.g., with smaller contact points).
- Specialized Tools & Techniques: Utilizing appropriate tools (manual, CNC, wire EDM) and developing specific procedures for support removal based on geometry.
- Qualifizierte Techniker: Employing well-trained technicians with experience in handling delicate AM parts.
- Oberflächenveredelung: Post-removal finishing steps (blasting, blending) are needed to smooth areas where supports were attached.
4. Powder Contamination and Management:
- Herausforderung: Metal powders, especially reactive ones like titanium, can be contaminated by exposure to oxygen/humidity, cross-contamination with other metals, or improper handling. Contaminated powder can lead to defects and compromised material properties. Ensuring complete removal of unfused powder from complex geometries is also critical.
- Lösungen und bewährte Praktiken:
- Strict Powder Handling Protocols: Storing powder in controlled, inert environments; using dedicated equipment for specific materials; implementing rigorous sieving and testing procedures for virgin and recycled powder.
- Rückverfolgbarkeit: Maintaining batch traceability for all powder used.
- Design for Powder Removal: Incorporating drainage holes or channels in designs with internal cavities or lattices to facilitate powder removal via vibration, compressed air, etc.
- Cleaning Validation: Validating cleaning processes to ensure removal of all loose powder particles. CT scanning can sometimes be used to verify powder removal from internal channels.
5. Ensuring Biocompatibility and Cleanliness:
- Herausforderung: Beyond the inherent biocompatibility of Ti-6Al-4V ELI, the entire manufacturing process (printing, handling, machining, finishing, cleaning) must not introduce contaminants or surface conditions that could elicit an adverse biological response. Residual manufacturing materials (powder, cutting fluids, polishing compounds) are unacceptable.
- Lösungen und bewährte Praktiken:
- Materialzertifizierung: Using only medically certified Ti-6Al-4V ELI powder from qualified vendors.
- Validated Post-Processing: All post-processing steps, especially cleaning, must be rigorously validated using analytical techniques (e.g., TOC analysis, cytotoxicity testing) to demonstrate the removal of potential residues to safe levels.
- Cleanroom Environment: Performing final cleaning and packaging in a certified cleanroom environment.
- Biocompatibility Testing: Performing biocompatibility tests (according to ISO 10993 standards) on final, sterilized parts as part of the overall device validation.
6. Achieving Consistent Quality and Regulatory Compliance:
- Herausforderung: Consistently producing high-quality, patient-specific implants that meet all specifications and regulatory requirements demands a robust quality management system (QMS). Variability in machines, materials, or processes can impact outcomes.
- Lösungen und bewährte Praktiken:
- ISO 13485-Zertifizierung: Implementing and maintaining a QMS certified to ISO 13485 (Medical devices – Quality management systems) is fundamental for medical device manufacturers and key suppliers.
- Prozessvalidierung (IQ/OQ/PQ): Thoroughly validating all equipment (Installation Qualification), processes (Operational Qualification), and ensuring consistent product quality (Performance Qualification).
- Standard Operating Procedures (SOPs): Documenting and strictly adhering to SOPs for every step of the workflow.
- Operator Training: Ensuring all personnel involved are adequately trained.
- Change Control: Implementing strict change control procedures for any modifications to materials, processes, or equipment.
- Comprehensive Documentation: Maintaining detailed records (design history file, device master record, device history record) for traceability and regulatory submissions.
Navigating these challenges requires a combination of technological expertise, meticulous process control, and a deep commitment to quality. Partnering with knowledgeable and experienced organizations like Met3dp, who provide not just equipment and materials but also comprehensive application support and understand the intricacies of the entire additive manufacturing workflow for medical devices, can significantly de-risk the process for manufacturers and ensure that wholesale distributors and healthcare providers receive safe, effective, and reliable custom bone plates.
Choosing the Right Metal 3D Printing Service Provider for Medical Devices: A Procurement Guide
Selecting the right manufacturing partner is arguably one of the most critical decisions when sourcing custom 3D printed non-load-bearing bone plates. The unique demands of medical device manufacturing, particularly for implants, mean that not just any additive manufacturing bureau will suffice. Procurement managers, engineers, and supply chain specialists within medical device companies, wholesale distributors, and hospital networks need a rigorous evaluation process to identify suppliers capable of consistently delivering safe, effective, and compliant products. Choosing the wrong partner can lead to delays, quality issues, regulatory non-compliance, and potentially compromise patient safety.
Here are key criteria to consider when evaluating potential metal AM service providers for medical device components like Ti-6Al-4V ELI bone plates:
1. Certifications and Regulatory Compliance:
- ISO 13485:2016: This is the nicht verhandelbar international standard for Quality Management Systems (QMS) for medical device manufacturers and suppliers. Certification demonstrates that the provider has established and maintains a QMS capable of consistently meeting customer and applicable regulatory requirements. Lack of this certification should be an immediate disqualifier for implant manufacturing.
- FDA Registration / Compliance (if applicable): If the final medical device will be marketed in the United States, the manufacturing partner may need to be registered with the FDA and comply with its Quality System Regulation (QSR) under 21 CFR Part 820. Understanding the supplier’s experience with FDA requirements is crucial.
- Other Certifications (e.g., ISO 9001): While ISO 13485 is primary, ISO 9001 (general QMS) can indicate a broader commitment to quality principles.
2. Proven Medical Device Experience:
- Erfolgsbilanz: Look for providers with demonstrable experience specifically in manufacturing orthopedic implants, preferably titanium components produced via AM. Ask for case studies, references (while respecting confidentiality), and evidence of successfully produced parts that have met clinical requirements.
- Understanding of Medical Needs: The provider should understand the unique challenges and critical nature of medical implants, including biocompatibility requirements, sterilization compatibility, and the importance of anatomical accuracy. They should speak the language of medical device development.
3. Material Expertise and Traceability:
- Material Sourcing: Where do they source their Ti-6Al-4V ELI powder? Is it from reputable suppliers adhering to medical standards (e.g., ASTM F136)? Do they perform incoming material inspection and testing?
- Powder Handling & Management: Do they have strict protocols for powder storage, handling, sieving, recycling, and testing to prevent contamination and ensure consistency? Cross-contamination between different metal types is a major risk that must be controlled.
- Full Material Traceability: The provider must demonstrate end-to-end traceability, linking the final implant back to the specific powder batch used, processing parameters, operator logs, and quality checks. This is essential for investigation should any issues arise.
4. Technological Capabilities and Process Control:
- AM Technology: Do they utilize appropriate, well-maintained AM technology (e.g., SLM, SEBM)? Different technologies have nuances. For instance, SEBM technology, like that developed by Met3dp, often operates at higher temperatures, which can reduce residual stress in titanium parts, potentially simplifying post-processing. Understand their machine park, maintenance schedules, and calibration procedures.
- Prozess-Validierung: Have they validated their printing processes (IQ/OQ/PQ) specifically for Ti-6Al-4V ELI medical applications? This provides confidence in the consistency and repeatability of their manufacturing output.
- Parameter Control: Do they demonstrate robust control over critical process parameters (laser/beam power, scan speed, layer thickness, atmosphere control, temperature)?
5. Umfassende Nachbearbeitungsmöglichkeiten:
- In-House vs. Outsourced: Does the provider handle critical post-processing steps (heat treatment/HIP, support removal, machining, finishing, cleaning) in-house, or do they rely on qualified external partners? If outsourced, how do they manage and qualify these vendors?
- Validierte Prozesse: Crucially, are these post-processing steps, especially cleaning and heat treatment/HIP, properly validated to meet medical device standards? Request evidence of validation protocols and reports. HIP capability is particularly important for ensuring maximum density and fatigue performance in critical implants.
- Finishing Options: Can they achieve the required surface finish specifications through controlled processes like bead blasting, tumbling, or electropolishing?
6. Ein solides Qualitätsmanagementsystem (QMS):
- Beyond Certification: Look deeper than just the ISO 13485 certificate. Assess the maturity and implementation of their QMS. This includes documentation control, record keeping, risk management practices, corrective and preventive action (CAPA) procedures, internal audits, and management review processes.
- Inspektion und Prüfung: What are their capabilities for dimensional inspection (CMM, 3D scanning), surface analysis, material testing, and potentially NDT (X-ray/CT)? How are inspection results documented and tied to part traceability?
7. Design and Engineering Support:
- DfAM-Fachwissen: Does the provider offer Design for Additive Manufacturing support? Can they collaborate effectively with your surgical or engineering team to optimize designs for printability, functionality, and cost-effectiveness while meeting clinical needs?
- Software and Workflow: What software tools do they use? How do they manage design iterations and ensure version control for patient-specific files?
8. Capacity, Lead Time, and Scalability:
- Durchlaufzeit: Can they consistently meet the lead times required for clinical scheduling or supply chain demands? Understand their typical lead times for custom Ti-6Al-4V ELI plates and their process for handling expedited orders (if needed, and at what cost).
- Produktionskapazität: Do they have sufficient machine capacity and personnel to handle your current and potential future volume requirements, especially relevant for wholesale distributors planning larger batch orders or ongoing supply contracts?
- Skalierbarkeit: Can they scale production up or down based on demand fluctuations?
9. Communication, Transparency, and Partnership:
- Reaktionsfähigkeit: Are they responsive to inquiries and requests for quotation (RFQ)? Is communication clear and professional?
- Transparenz: Are they open about their processes, capabilities, and limitations? Is their pricing structure clear and understandable?
- Kollaborativer Ansatz: Do they view the relationship as a true partnership focused on mutual success and patient safety, rather than just a transactional one?
10. Confidentiality and Intellectual Property (IP) Protection:
- Data Security: How do they ensure the confidentiality and security of sensitive patient data (scan data) and proprietary implant designs? Non-disclosure agreements (NDAs) are standard practice.
Supplier Audit:
For critical implant manufacturing, a thorough supplier audit is highly recommended. This involves visiting the facility (or conducting a detailed remote audit), reviewing documentation, interviewing key personnel, and observing processes firsthand to verify their capabilities and compliance against these criteria.
Choosing the right metal AM service provider is a strategic decision. It requires careful due diligence beyond just comparing price quotes. Focusing on quality, compliance, expertise, and a collaborative approach will lead to a reliable supply of high-performance, patient-specific bone plates that meet the demanding standards of the medical industry.
Understanding Cost Factors and Lead Times for Custom Bone Plates: Wholesale and Production Insights
One of the primary considerations for medical device companies, hospital procurement departments, and wholesale distributors exploring custom 3D printed bone plates is understanding the associated costs and production timelines. While offering significant clinical advantages, patient-specific manufacturing inherently differs from the economics of mass-produced standard implants. A clear grasp of the cost drivers and lead time components is essential for accurate budgeting, surgical planning, and effective supply chain management.
Cost Factors for Custom 3D Printed Bone Plates:
The final price of a custom Ti-6Al-4V ELI bone plate is influenced by a complex interplay of factors. It’s rarely a simple per-unit cost like off-the-shelf items. Key drivers include:
- Design Complexity and Engineering Time:
- Patient-Specific Design: Creating a unique design for each patient requires skilled engineering time for image segmentation, CAD modeling, potential FEA simulation, and design reviews with the surgeon. This upfront engineering effort contributes significantly to the cost of a single custom plate.
- Geometric Complexity: Intricate features like complex curvatures, thin walls, integrated lattice structures, or numerous angled screw holes increase design time and potentially printing/post-processing difficulty, impacting cost.
- Part Size and Volume:
- Materialverbrauch: Larger or thicker plates require more Ti-6Al-4V ELI powder, a relatively expensive raw material.
- Build Volume Occupation: The space the part occupies within the AM machine’s build chamber (its bounding box) influences how many parts can be printed simultaneously, affecting machine utilization cost. Larger parts take up more space and potentially require longer build times.
- Additive Manufacturing (Printing) Time:
- Maschine Stundensatz: AM machines represent a significant capital investment and have operational costs (energy, inert gas, maintenance). Pricing is often linked to the time required to print the part(s), which depends on the part’s height (number of layers), volume, and the chosen process parameters (e.g., layer thickness, scan speed).
- Nesting-Effizienz: For batch production (relevant for distributors stocking semi-custom ranges or research batches), how efficiently multiple parts can be “nested” within a single build significantly impacts per-part print cost.
- Nachbearbeitungsintensität:
- Heat Treatment / HIP: Stress relief and particularly Hot Isostatic Pressing (HIP) require specialized furnace equipment and add significant time and cost but are crucial for quality.
- Unterstützung bei der Entfernung: Complex parts with extensive or hard-to-reach supports require more manual labor or specialized techniques (e.g., EDM), increasing cost.
- Oberflächenveredelung: The level of surface finishing required (e.g., basic bead blast vs. electropolishing or manual polishing) directly impacts labor and processing time.
- Bearbeitungen: Any required CNC machining for critical features adds setup time, machine time, and labor cost.
- Reinigung und Inspektion: Rigorous, validated cleaning processes and thorough quality inspection (visual, dimensional, NDT) contribute to the overall cost but are essential for medical devices.
- Materialkosten:
- Ti-6Al-4V ELI Powder: Medical-grade titanium powder meeting stringent standards (e.g., ASTM F136) is inherently costly due to the raw materials and specialized production processes (like PREP or high-purity GA). Powder quality directly impacts final part quality.
- Quality Assurance and Regulatory Overhead:
- QMS Maintenance: Operating under a certified ISO 13485 QMS involves ongoing costs for documentation, audits, training, and compliance activities, which are factored into the pricing.
- Validation Efforts: Validating processes requires significant upfront investment.
- Batch Size and Order Volume:
- Single Patient vs. Batch: Producing a single, unique patient-specific plate incurs higher per-unit costs due to dedicated design time and machine setup.
- Mengenrabatte: For wholesale distributors or hospitals ordering larger batches of standardized (or semi-custom) designs, economies of scale can be realized through more efficient nesting, streamlined workflows, and amortized setup costs, leading to lower per-part pricing. Discuss volume pricing tiers with potential suppliers.
- Expedite Fees:
- Urgent Orders: Requesting faster-than-standard lead times often incurs expedite fees to cover overtime, re-prioritization of machine schedules, and potentially dedicated processing.
Lead Time Components and Typical Durations:
Lead time refers to the total time elapsed from order placement (or design finalization) to shipment of the finished, inspected implant. It’s crucial for surgical scheduling and inventory planning.
- Key Stages Contributing to Lead Time:
- Design Phase (if applicable): Segmentation, CAD design, review/approval cycles (can take hours to days, depending on complexity and responsiveness).
- Order Processing & Scheduling: Placing the job in the production queue (can range from immediate to several days, depending on supplier workload).
- Build Setup & Printing: Preparing the build file, setting up the machine, and the actual AM print time (typically 12 hours to several days, depending on build height and part density).
- Abkühlung und Entfettung: Allowing the build chamber to cool, removing the build plate, and carefully removing unfused powder (several hours).
- Heat Treatment / HIP: Furnace cycle time, including heating, soaking, cooling (can take 1-2 days, including setup).
- Part Removal & Support Removal: Separating parts from the build plate and removing supports (hours to days, depending on complexity and method).
- Machining & Surface Finishing: Time required for any necessary machining and surface treatments (hours to days).
- Cleaning & Drying: Validated cleaning cycles (several hours).
- Qualitätsinspektion: Dimensional checks, surface verification, documentation review (hours to a day).
- Packaging & Shipping: Final preparation for shipment.
- Typische Gesamtvorlaufzeiten:
- Highly Variable: It’s difficult to give a single definitive answer, as lead times depend heavily on the factors above (complexity, batch size, supplier workload, required post-processing).
- General Range (Custom Plate): For a single patient-specific Ti-6Al-4V ELI bone plate requiring standard post-processing including HIP, lead times often range from 1 bis 4 Wochen after final design approval.
- Eiliger Dienst: May reduce lead times significantly but comes at a premium cost.
- Batch Production: Lead times for larger batches may be longer overall but result in a lower per-part lead time average once production is streamlined.
Managing Expectations:
Clear communication between the medical device company/hospital and the AM service provider is vital. Understanding the breakdown of costs and the factors influencing lead time allows for better planning. When requesting quotes (RFQs), provide detailed information (CAD models if possible, material specs, required tolerances, surface finish, batch size, desired timeline) to receive accurate pricing and lead time estimates. Consider the “total cost of ownership,” factoring in potential savings from reduced OR time or improved outcomes, not just the per-part implant cost, when comparing custom AM plates to traditional options.
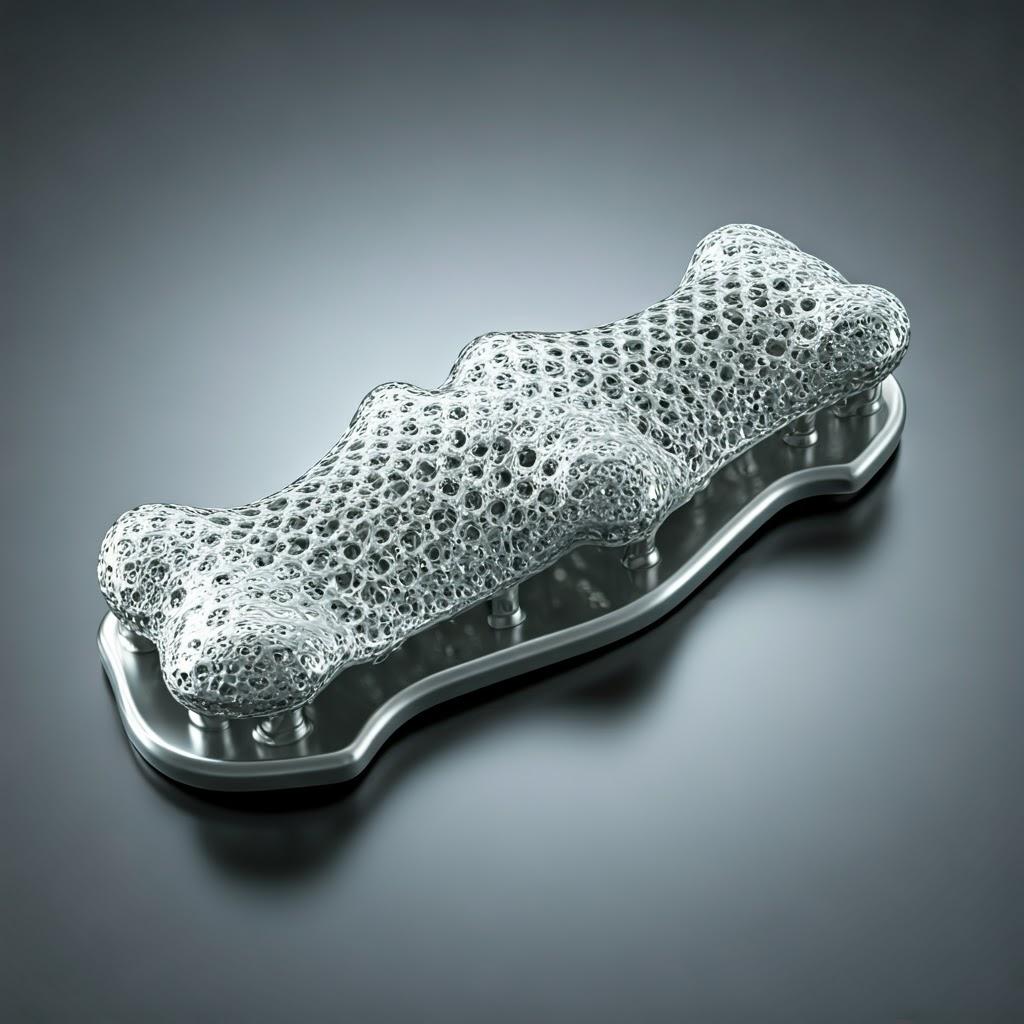
Frequently Asked Questions (FAQ) about 3D Printed Titanium Bone Plates
As metal additive manufacturing becomes more prevalent in orthopedics, surgeons, procurement specialists, engineers, and even patients often have questions about custom 3D printed titanium bone plates. Here are answers to some common inquiries:
1. Is 3D printed Ti-6Al-4V ELI safe for long-term implantation in the human body?
Antwort: Yes, absolutely. When manufactured correctly, 3D printed Ti-6Al-4V ELI (Grade 23) is considered very safe for long-term implantation. Here’s why:
- Material Biocompatibility: Ti-6Al-4V ELI itself has a long and successful history in medical implants due to its excellent biocompatibility, corrosion resistance, and favorable mechanical properties. It conforms to stringent international standards like ASTM F136 (“Standard Specification for Wrought Titanium-6Aluminum-4Vanadium ELI (Extra Low Interstitial) Alloy for Surgical Implant 1 Applications”). While this standard originally pertained to wrought materials, its chemical composition and biocompatibility benchmarks are the foundation. Additive manufacturing standards specific to Ti-6Al-4V (like ASTM F3001 for PBF) ensure AM parts meet similar criteria. 1. steelplates.in steelplates.in
- Validierte Prozesse: The safety hinges on using high-purity, certified medical-grade powder and employing rigorously validated manufacturing processes. This includes optimized printing parameters to achieve near-full density, mandatory heat treatments (like stress relief and HIP) to ensure appropriate microstructure and mechanical integrity, and validated cleaning procedures to remove any residual contaminants.
- Regulatory Oversight: Medical devices, including 3D printed implants, are subject to strict regulatory oversight (e.g., by the FDA in the US, or under the MDR in Europe). Manufacturers must demonstrate safety and efficacy through extensive testing, process validation, and adherence to quality management systems like ISO 13485.
- Clinical Evidence: There is a growing body of clinical evidence demonstrating the successful use of 3D printed Ti-6Al-4V ELI implants in various orthopedic applications, including craniofacial reconstruction and fracture fixation, with excellent patient outcomes.
In summary, the material itself is proven safe, and when combined with controlled, validated additive manufacturing and post-processing workflows, 3D printed Ti-6Al-4V ELI implants meet the high safety standards required for medical use.
2. How does the cost of a custom 3D printed bone plate compare to a standard, off-the-shelf plate?
Antwort: Comparing costs requires looking beyond the price tag of the implant itself.
- Per-Implant Cost: A custom, patient-specific 3D printed bone plate will generally have a higher per-implant manufacturing cost than a mass-produced, standard off-the-shelf plate. This is due to the dedicated design engineering time, unique machine setup for potentially single-piece production, and often more complex geometry requiring careful post-processing.
- Total Procedure Cost & Value: The value proposition of custom plates often lies in potential savings across the entire treatment episode:
- Reduced Operating Room (OR) Time: Eliminating the need for surgeons to manually bend standard plates during surgery can significantly shorten OR time. OR time is extremely expensive (costing many dollars/euros per minute), so even a 20-30 minute reduction can lead to substantial cost savings.
- Improved Surgical Outcomes: The precise anatomical fit can lead to more stable fixation, potentially faster healing, reduced risk of complications (like malunion or infection), and potentially fewer revision surgeries down the line. Avoiding complications and revisions represents significant long-term cost savings and improved patient quality of life.
- Reduzierte Bestände: Hospitals and distributors can reduce costs associated with managing large inventories of standard plates in numerous sizes, minimizing waste from expired or obsolete stock.
- Complex Cases: For highly complex cases where standard plates offer a poor solution, custom plates may be the only viable option or may significantly simplify an otherwise challenging procedure, providing value that’s hard to quantify solely by implant cost.
Conclusion: While the initial implant cost is higher, the overall value derived from reduced OR time, better outcomes, and inventory optimization often makes custom 3D printed plates a cost-effective solution, especially for complex non-load-bearing applications.
3. What information is typically needed to order a custom 3D printed bone plate?
Antwort: Ordering a patient-specific bone plate requires specific inputs to enable the design and manufacturing process:
- High-Resolution Medical Imaging Data: This is the foundation. A high-quality CT scan (Computed Tomography) of the patient’s relevant anatomy is usually required. The scan should follow specific protocols regarding slice thickness (typically 1mm or less) and resolution to ensure sufficient detail for accurate 3D model reconstruction. The data is typically provided in DICOM format. MRI data might supplement in some cases.
- Surgical Plan / Prescription: Information from the surgeon outlining the clinical need, the location and type of fracture or defect, desired fixation points, any critical anatomical structures to avoid, and specific requirements for the plate (e.g., desired profile, need for porous structures).
- Collaborative Design Process: Usually, there’s an interactive process between the surgeon and the design engineer (at the medical device company or the AM service provider). This involves reviewing the initial anatomical model, proposing a plate design, virtually simulating screw placement, making necessary revisions based on surgeon feedback, and final design approval by the surgeon before manufacturing commences.
- Patient Demographics (Anonymized if possible): Basic information might be needed for identification and traceability through the production process, always adhering to strict data privacy regulations (e.g., HIPAA, GDPR). Unique identifiers are often used.
- Purchase Order / Commercial Agreement: Standard commercial documentation outlining the terms, pricing, and delivery requirements.
Ensuring clear communication and providing complete, high-quality input data is crucial for the efficient and accurate production of a custom implant.
Conclusion: The Future of Orthopedic Implants with Metal 3D Printing and Met3dp
The integration of metal additive manufacturing into orthopedic surgery, particularly for non-load-bearing applications like custom bone plates, marks a significant leap forward in patient care. By harnessing the power of 3D printing with advanced materials like Ti-6Al-4V ELI, we are moving beyond the limitations of traditional, standardized implants towards a future of truly personalized medicine.
The advantages are compelling and clear:
- Unmatched Anatomical Fit: Patient-specific plates derived from CT data offer superior conformity, leading to enhanced stability, potentially faster healing, and improved functional and aesthetic outcomes, especially in complex craniofacial and maxillofacial reconstructions.
- Design Freedom for Optimal Function: AM enables the creation of intricate geometries, including optimized shapes, variable thickness, and porous structures designed to encourage osseointegration, leading to better long-term biological fixation.
- Operational Efficiencies: Pre-contoured custom plates significantly reduce intraoperative time spent bending implants, saving valuable OR time, reducing anesthesia exposure, and potentially lowering overall procedural costs.
- Solutions for Complex Cases: This technology provides effective solutions for challenging anatomical variations or defects where standard implants fall short.
However, realizing these benefits requires a commitment to excellence at every stage. It demands high-quality input data, meticulous design based on DfAM principles, the use of certified medical-grade materials like Ti-6Al-4V ELI, and rigorously controlled and validated manufacturing processes – from printing through heat treatment, HIP, finishing, and cleaning. The selection of a capable and compliant manufacturing partner, one with proven medical device expertise and a robust ISO 13485 certified quality system, is paramount.
The future of orthopedic implants undoubtedly involves greater personalization, leveraging digital workflows and advanced manufacturing. We envision a landscape where surgeons can routinely order custom implants tailored precisely to each patient’s unique needs, leading to more predictable and successful surgeries. Metal 3D printing is the core enabling technology driving this transformation.
Companies like Met3dp are at the forefront of this evolution. With deep expertise spanning both the production of high-performance, spherical metal powders optimized for AM and the development of industry-leading Selective Electron Beam Melting (SEBM) printing systems, Met3dp provides comprehensive solutions for the medical device industry. Our commitment to quality, innovation, and process control ensures that our partners have access to the materials and technology needed to produce safe, reliable, and effective 3D printed medical implants. We partner with medical device manufacturers, research institutions, and wholesale suppliers to accelerate the adoption of additive manufacturing and unlock its full potential.
To learn more about how Met3dp’s advanced metal powders and SEBM printing solutions can empower your organization’s journey into the future of personalized orthopedic implants, please Kontaktieren Sie uns to discuss your specific needs. Let’s build the future of medical device manufacturing together.
Teilen auf
Facebook
Twitter
LinkedIn
WhatsApp
E-Mail
MET3DP Technology Co., LTD ist ein führender Anbieter von additiven Fertigungslösungen mit Hauptsitz in Qingdao, China. Unser Unternehmen ist spezialisiert auf 3D-Druckgeräte und Hochleistungsmetallpulver für industrielle Anwendungen.
Fragen Sie an, um den besten Preis und eine maßgeschneiderte Lösung für Ihr Unternehmen zu erhalten!
Verwandte Artikel
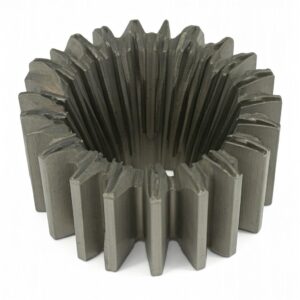
Hochleistungs-Düsenschaufelsegmente: Revolutionierung der Turbineneffizienz mit 3D-Metalldruck
Mehr lesen "
Mai 13, 2025
Keine Kommentare
Über Met3DP
Aktuelles Update
Unser Produkt
KONTAKT US
Haben Sie Fragen? Senden Sie uns jetzt eine Nachricht! Wir werden Ihre Anfrage mit einem ganzen Team nach Erhalt Ihrer Nachricht bearbeiten.
Holen Sie sich Metal3DP's
Produkt-Broschüre
Erhalten Sie die neuesten Produkte und Preislisten
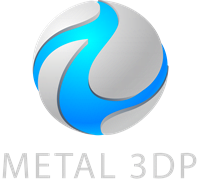
Metallpulver für 3D-Druck und additive Fertigung
UNTERNEHMEN
PRODUKT
cONTACT INFO
- Qingdao Stadt, Shandong, China
- [email protected]
- [email protected]
- +86 19116340731