Lightweight Satellite Brackets via Aluminum 3D Printing
Inhaltsübersicht
Introduction: The Critical Role of Lightweighting in Satellite Brackets
Satellites represent the pinnacle of human engineering, operating autonomously in the harsh environment of space to perform critical functions ranging from global communications and navigation to Earth observation and scientific discovery. Every component within these sophisticated machines is meticulously designed and rigorously tested to ensure reliability and performance under extreme conditions, including launch vibrations, thermal cycling, and radiation exposure. Among the essential, yet often overlooked, components are satellite brackets. These structural elements, while seemingly simple, perform the vital task of mounting, securing, and aligning various subsystems within the satellite bus or payload module. They are the unsung heroes ensuring that sensitive avionics, deployable appendages like solar arrays and antennas, propulsion tanks, scientific instruments, and intricate wiring harnesses remain precisely positioned and structurally sound throughout the mission lifecycle.
However, in the realm of space exploration and satellite deployment, one factor reigns supreme: mass. Every single gram launched into orbit carries a significant cost penalty, often measured in thousands, or even tens of thousands, of US dollars per kilogram depending on the launch vehicle and target orbit (Low Earth Orbit, Geostationary Orbit, etc.). This economic reality places an immense pressure on satellite designers and manufacturers to minimize the mass of every component without compromising structural integrity or functionality. This relentless pursuit of mass reduction is known as Leichtbau, and it is a fundamental principle guiding satellite design. Reducing the mass of structural components like brackets directly translates to lower launch costs, potentially allowing for more payload capacity (e.g., additional instruments or fuel for longer missions), or enabling the use of smaller, less expensive launch vehicles. Furthermore, reducing overall satellite mass can improve maneuverability and reduce the fuel required for orbital adjustments.
Traditionally, engineers relied on materials like aluminum alloys, known for their favorable strength-to-weight ratio, and manufacturing processes like CNC (Computer Numerical Control) machining to create satellite brackets. While effective, machining involves starting with a solid block of material and removing excess material (subtractive manufacturing), which can lead to significant material waste and limitations in geometric complexity, often preventing truly optimized, lightweight designs. Other methods like casting might be used for complex shapes but often involve tooling costs, longer lead times, and potentially lower material properties compared to wrought or forged materials.
Eingeben Additive Fertigung von Metall (AM)gemeinhin als Metall bekannt 3D-Druck. This transformative technology offers a paradigm shift in how components like satellite brackets are designed and produced. Instead of removing material, AM builds parts layer by layer directly from a digital model using metal powders. This additive approach unlocks unprecedented design freedom, enabling the creation of highly complex, organically shaped, and topologically optimized structures that were previously impossible or prohibitively expensive to manufacture. Specifically for satellite brackets, Aluminium-3D-Druck, particularly using processes like Laser Powder Bed Fusion (LPBF), allows engineers to leverage the inherent lightweight nature of aluminum while exploiting AM’s capabilities to drastically reduce component mass far beyond what’s achievable with traditional methods. This synergy between advanced aluminum alloys and additive manufacturing techniques is revolutionizing the design and production of satellite structural components, paving the way for lighter, more capable, and more cost-effective satellites. This article delves into the specifics of utilizing aluminum 3D printing for manufacturing lightweight satellite brackets, exploring the applications, benefits, materials, design considerations, and supplier selection criteria crucial for aerospace engineers and procurement managers operating in this demanding sector.
Applications: Where are 3D Printed Aluminum Satellite Brackets Used?
The versatility of aluminum additive manufacturing allows for the creation of bespoke brackets tailored to a vast array of functions within a satellite’s architecture. The ability to consolidate multiple functions into a single, complex part while minimizing mass makes 3D printed aluminum brackets particularly attractive for numerous satellite subsystem mounting tasks. Here’s a closer look at some key application areas:
- Avionics and Electronics Housing/Mounting: Satellites are packed with sensitive electronic components, including onboard computers, communication transponders, power distribution units, and data handling systems. These avionics brackets must securely hold these delicate components, protecting them from the intense vibrations and g-forces experienced during launch. Furthermore, electronics generate heat, and brackets can be designed with integrated thermal management features, such as complex internal channels for heat pipes or optimized geometries that enhance conductive heat transfer to the satellite’s main structure or radiators. 3D printing allows for highly customized enclosures and mounts that conform perfectly to the electronic boxes, minimizing volume and mass while maximizing structural support and thermal efficiency.
- Solar Array and Antenna Deployment Mechanisms: Large deployable structures like solar panels and communication antennas are critical for satellite operation but must be stowed securely during launch and deployed reliably once in orbit. Brackets play a crucial role in the hinges, latches, and support structures of these solar array deployment mechanisms and antenna pointing systems. 3D printed aluminum brackets, particularly those made from high-strength alloys like Scalmalloy®, can provide the necessary stiffness and strength to handle deployment loads while being significantly lighter than traditionally machined counterparts. The design freedom allows for complex kinematics and integrated features within the bracket structure itself.
- Propulsion System Components: Brackets are needed to mount fuel tanks, propellant lines, valves, and thrusters. These components must withstand significant pressure variations, thermal cycles, and vibrations, especially during engine firing. 3D printed aluminum brackets can be topologically optimized to handle these specific load paths, ensuring secure mounting with minimal mass. The ability to create complex geometries also facilitates efficient routing of propellant lines and integration of sensors.
- Instrument and Sensor Mounting: Scientific instruments, Earth observation sensors, cameras, and star trackers require extremely stable and precise mounting platforms. Any minor shift or vibration can compromise data quality. 3D printed brackets can be designed with high stiffness-to-weight ratios and customized geometries to ensure precise alignment and minimize thermally induced distortions. Part consolidation can also reduce the number of interfaces, further enhancing stability.
- Optical Bench Supports: For satellites carrying telescopes or optical communication systems, the alignment of mirrors, lenses, and detectors is paramount. Brackets forming part of the optical bench must provide exceptional stability and minimal thermal expansion. While materials like Invar are often used for ultimate stability, lightweight aluminum AM brackets can be employed in supporting structures, sometimes incorporating complex lattice designs to achieve high stiffness with very low mass.
- Waveguide and Coaxial Cable Routing: Efficiently routing RF waveguides and electrical cabling throughout the satellite is essential. Custom 3D printed brackets can provide secure clamping and precise routing paths, often conforming to complex structural contours. This prevents damage from vibration and simplifies assembly. These brackets can be designed with smooth, rounded edges to prevent cable chafing.
- Secondary Structural Supports: Beyond mounting specific equipment, 3D printed brackets are used as general secondary structural elements, connecting panels, reinforcing joints, and transferring loads within the satellite bus structure. Topology optimization is heavily employed here to create highly efficient, load-bearing structures that add minimal weight.
Beyond Satellites: Cross-Industry Relevance
The principles and benefits observed in satellite applications readily translate to other industries where weight, performance, and complexity are critical factors:
- Unmanned Aerial Vehicles (UAVs / Drones): Lightweighting is crucial for extending flight time and increasing payload capacity. 3D printed aluminum brackets are used for mounting motors, sensors, batteries, and landing gear.
- High-Performance Automotive (Motorsport, Electric Vehicles): Reducing weight improves acceleration, handling, and efficiency. AM brackets find use in engine/powertrain mounting, suspension components, and mounting electronic control units (ECUs).
- Medizinische Geräte: While different materials are often used (like Titanium), the principle of creating complex, lightweight, patient-specific or device-specific brackets for implants or external devices leverages AM’s capabilities.
- Robotics and Industrial Automation: Custom brackets for mounting grippers, sensors, and actuators on robotic arms benefit from the design freedom and potential weight savings offered by AM.
In essence, wherever a component is needed to connect, support, mount, or align other parts, and where reducing mass while maintaining or enhancing performance is a key objective, 3D printed aluminum brackets present a compelling manufacturing solution, especially for complex designs or low-to-medium volume production runs typical in aerospace component manufacturing and related high-tech fields.
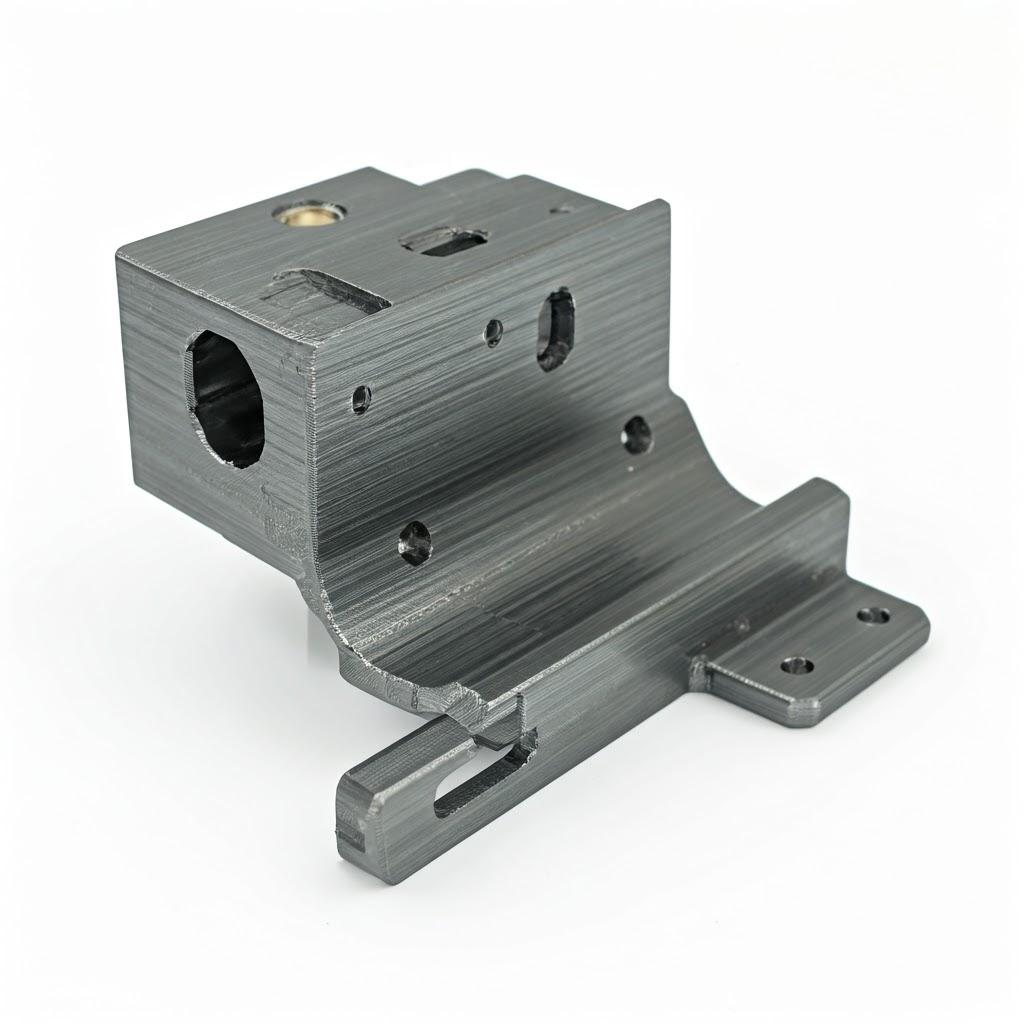
Why Metal 3D Printing for Satellite Brackets? Unlocking Performance Gains
The decision to adopt metal additive manufacturing, specifically Laser Powder Bed Fusion (LPBF), for producing satellite brackets stems from a confluence of compelling advantages over traditional manufacturing techniques. These benefits directly address the core challenges of aerospace engineering: maximizing performance while minimizing weight and cost, and accelerating development timelines. For procurement managers and engineers evaluating production methods, understanding these advantages is key to making informed sourcing decisions.
1. Unprecedented Design Freedom and Complexity:
- Breaking Traditional Constraints: Conventional manufacturing methods, like CNC machining, are inherently limited by tool access and the subtractive nature of the process. Complex internal features, undercuts, and organic shapes are often difficult, time-consuming, or impossible to create. Metal AM, building parts layer-by-layer, removes these constraints.
- Topologie-Optimierung: This is arguably the most significant driver for AM in structural components. Specialized software analyzes the load paths and stresses a bracket will experience in operation and then algorithmically removes material from non-critical areas, leaving behind an optimized, often organic-looking structure that meets all performance requirements with the minimum possible mass. This approach can yield weight savings of 30-70% or even more compared to conventionally designed and manufactured parts, directly impacting launch costs and satellite performance. This is a core tenet of topology optimization aerospace Anwendungen.
- Gitterförmige Strukturen: AM enables the incorporation of complex internal lattice structures. These engineered micro-architectures can significantly enhance stiffness-to-weight ratios, provide vibration damping characteristics, or facilitate heat transfer, all within the bracket’s design envelope.
- Generative Design: Going beyond topology optimization, generative design tools can autonomously create hundreds or thousands of design variations based on predefined constraints (loads, material, manufacturing process, keep-out zones), allowing engineers to explore a wider design space and discover novel, high-performance solutions.
2. Part Consolidation:
- Reducing Assembly Complexity: Traditionally, a complex bracket assembly might consist of multiple individual pieces (machined plates, bent sheet metal, fasteners). Each part adds weight, requires assembly time, and introduces potential failure points at joints and interfaces.
- Enhanced Reliability: Metal AM allows engineers to consolidate these multiple components into a single, monolithic 3D printed part. This drastically reduces the number of fasteners (bolts, rivets), seals, and interfaces, leading to part consolidation benefits such as lower assembly labor costs, reduced part count and inventory management, lighter overall weight, and significantly improved structural integrity and reliability by eliminating potential failure modes associated with joints.
3. Rapid Prototyping and Accelerated Development:
- Speeding Up Iteration: In the fast-paced world of satellite development, the ability to quickly iterate on designs is invaluable. Traditional manufacturing often involves long lead times for tooling (casting) or complex machining setups. With AM, a design modification can be implemented in CAD, and a new prototype can often be printed within days or weeks, not months.
- Faster Qualification: This rapid prototyping capability allows for quicker physical testing and validation of bracket designs, significantly accelerating the rapid prototyping space hardware cycle and reducing the overall time-to-flight for new satellite missions or components. Functional prototypes can be produced in the target material (AlSi10Mg or Scalmalloy®), providing highly relevant test data early in the development process.
4. Reduced Material Waste:
- Additiv vs. Subtraktiv: CNC machining starts with a solid block or billet of material and cuts away the excess. For complex geometries, the amount of material removed (and wasted as chips or scrap) can be substantial, sometimes exceeding 80-90% of the initial block (the “buy-to-fly” ratio).
- Efficient Material Usage: LPBF uses only the material necessary to build the part and its support structures. While some powder is used for supports and some cannot be fully recycled indefinitely, the overall material utilization is significantly better than subtractive methods, especially for complex, lightweighted designs. This is becoming increasingly important from both cost and sustainability perspectives.
5. Suitability for Low-to-Medium Volume Production:
- Beseitigung von Werkzeugen: Processes like casting or injection molding require expensive upfront investment in molds or dies, making them economical only for high production volumes. Metal AM requires no part-specific tooling.
- Kosteneffiziente Anpassung: Dies macht LPBF aluminum printing highly cost-effective for the production volumes typically associated with satellites and other aerospace applications, where only a few or perhaps a few dozen identical brackets might be needed per mission or platform. It also allows for economical production of customized bracket variations for different satellite configurations.
Leveraging the Technology with Expertise:
While the benefits are clear, successfully implementing 3D-Druck von Metall requires specialized knowledge in design for AM (DfAM), materials science, process parameter control, and post-processing. Partnering with an experienced Metall-AM-Servicebüro or solutions provider is often crucial. Companies like Met3dp, with deep expertise in additive manufacturing technologies and high-performance materials, provide not just printing services but also comprehensive solutions, including design support and material guidance, ensuring that the full potential of AM is realized for critical components like satellite brackets. Their focus on industry-leading equipment and advanced powder manufacturing ensures the reliability and quality demanded by the aerospace sector.
Recommended Materials: AlSi10Mg and Scalmalloy® for Space Applications
Choosing the right material is paramount for designing satellite brackets that meet stringent performance, weight, and environmental requirements. Aluminum alloys are favored for their inherent low density, good thermal conductivity, and relative ease of processing via Laser Powder Bed Fusion (LPBF). Within the aluminum family, two alloys stand out for 3D printed satellite applications: AlSi10Mg and Scalmalloy®. Understanding their distinct properties and benefits is crucial for material selection.
AlSi10Mg: The Workhorse Aluminum Alloy
AlSi10Mg is one of the most common and well-characterized aluminum alloys used in metal additive manufacturing. It’s essentially a casting alloy adapted for LPBF, containing approximately 9-11% Silicon and 0.2-0.45% Magnesium.
- Key Properties & Benefits:
- Gutes Verhältnis von Stärke zu Gewicht: While not the highest strength aluminum alloy available, it offers a respectable strength level, particularly after appropriate heat treatment (typically T6), combined with low density (≈2.67g/cm3). This makes it suitable for a wide range of moderately loaded structural applications.
- Ausgezeichnete Wärmeleitfähigkeit: The high silicon content contributes to good thermal conductivity (≈100−140W/m⋅K depending on heat treatment), which is highly advantageous for brackets needing to dissipate heat from mounted electronics or other components.
- Gute Korrosionsbeständigkeit: AlSi10Mg exhibits good resistance to atmospheric corrosion.
- Processability & Cost-Effectiveness: It is relatively easy to process using LPBF, with well-established parameters available on many commercial machines. The powder is also generally less expensive than higher-performance alloys like Scalmalloy®.
- Schweißeignung: Although less relevant for monolithic AM parts, it possesses reasonable weldability, which can be useful if post-fabrication joining is required.
- Nachbearbeiten: It responds well to standard post-processing techniques, including stress relief, T6 heat treatment (solutionizing and artificial aging) to significantly improve strength and hardness, CNC machining, and surface finishing like anodizing or chemical conversion coating.
- Typische Anwendungen: Ideal for brackets with moderate structural loads, components where thermal management is a key consideration, complex geometries where manufacturability via AM is the primary driver, and applications where cost is a significant factor. Examples include avionics chassis, housings, general support structures, and heat sinks integrated into brackets.
Scalmalloy®: High-Performance Aluminum for Demanding Applications
Scalmalloy® is a patented high-performance aluminum-magnesium-scandium (Al-Mg-Sc) alloy specifically developed by APWorks (an Airbus subsidiary) for additive manufacturing. It pushes the boundaries of what’s achievable with aluminum alloys, offering properties that rival some titanium grades in specific aspects.
- Key Properties & Benefits:
- Außergewöhnliches Verhältnis von Stärke zu Gewicht: This is Scalmalloy®’s standout feature. It boasts significantly higher yield strength (≈450−520MPa) and ultimate tensile strength (≈500−580MPa) compared to AlSi10Mg (Yield Strength ≈230−300MPa post-T6), while maintaining a similar low density (≈2.67g/cm3). This allows for even greater weight savings in strength-critical applications.
- Excellent Ductility and Fatigue Strength: Unlike many high-strength aluminum alloys, Scalmalloy® retains good ductility (elongation ≈8−15%) and exhibits superior fatigue performance, making it suitable for components subjected to cyclic loading, vibrations, and dynamic stresses – common conditions for satellite components during launch and operation.
- High Specific Strength: Its combination of high strength and low density gives it a specific strength (strength divided by density) that surpasses many other aluminum and even some titanium alloys, making it ideal for aerospace lightweighting.
- Microstructure Stability at Elevated Temperatures: It retains its properties better at slightly elevated temperatures compared to standard aluminum alloys.
- Gute Schweißbarkeit und Korrosionsbeständigkeit: Similar to AlSi10Mg, it generally shows good weldability and corrosion resistance.
- Typische Anwendungen: Preferred for highly loaded structural brackets, components where fatigue life is critical, applications demanding the absolute minimum weight for a given strength requirement, and parts replacing heavier titanium components. Examples include primary load-bearing structures, deployment mechanism components, engine mounts, and brackets subjected to significant vibration or dynamic loads. The higher material and licensing costs are justified by the substantial performance gains.
Comparison Table: AlSi10Mg vs. Scalmalloy® for Satellite Brackets
Eigentum | AlSi10Mg (Typical T6 Heat Treated) | Scalmalloy® (Typical As-Built/Stress Relieved) | Einheit | Anmerkungen |
---|---|---|---|---|
Dichte | ≈2.67 | ≈2.67 | g/cm3 | Both offer significant weight savings over steel or titanium. |
Yield Strength (Rp0.2) | ≈230−300 | ≈450−520 | MPa | Scalmalloy® offers significantly higher strength. |
Ultimate Tensile Strength (Rm) | ≈330−430 | ≈500−580 | MPa | Scalmalloy® exhibits superior ultimate strength. |
Dehnung beim Bruch | ≈3−10 | ≈8−15 | % | Scalmalloy® generally offers better ductility at higher strength. |
Elastizitätsmodul | ≈70−75 | ≈70−76 | GPa | Similar stiffness for both materials. |
Wärmeleitfähigkeit | ≈100−140 | ≈120−150 | W/(m⋅K) | Both offer good thermal conductivity, slightly better for Scalmalloy®. |
Ermüdungsfestigkeit (R=-1) | Mäßig | Hoch | Comparative | Scalmalloy® excels in fatigue-critical applications. |
Maximale Betriebstemperatur | ≈100−150 | ≈150−200 | °C | Scalmalloy® retains strength better at slightly elevated temperatures. |
Relative Kosten | Unter | Höher | Comparative | AlSi10Mg is more cost-effective for less demanding applications. |
Hauptvorteil | Balanced Properties, Cost, Thermal | Maximum Strength-to-Weight, Fatigue Life | – | Choose based on primary design drivers. |
In Blätter exportieren
(Note: Properties are approximate and depend heavily on specific LPBF process parameters, build orientation, heat treatment, and testing conditions. Always refer to supplier datasheets for specific values.)
The Importance of Powder Quality and Supplier Expertise
Regardless of the alloy chosen, the quality and consistency of the metal powder used in the LPBF process are critical for achieving the desired mechanical properties and ensuring the reliability of the final satellite bracket. Defects in the powder (e.g., irregular shape, internal porosity, satellites, incorrect particle size distribution) can translate into defects in the printed part, potentially compromising its structural integrity.
This is where partnering with a knowledgeable aerospace metal powder supplier and AM service provider like Met3dp becomes essential. Met3dp employs industry-leading powder production technologies, including Gaszerstäubung and Plasma Rotating Electrode Process (PREP), to manufacture high-quality spherical metal powders.
- Gaszerstäubung: Utilizes unique nozzle and gas flow designs to produce metallic spheres with high sphericity and excellent flowability – crucial for uniform powder bed density in LPBF.
- PREP: Creates highly pure and spherical powders, often favored for reactive materials like titanium alloys but also applicable for ensuring premium quality in other metals.
Met3dp’s commitment extends beyond aluminum; their portfolio includes innovative alloys like TiNi, TiTa, TiAl, TiNbZr, CoCrMo, stainless steels, and superalloys, demonstrating a broad expertise in Met3dp Metall-Pulver suitable for demanding aerospace, medical, and industrial applications. This expertise in powder manufacturing, combined with their operation of industry-leading SEBM (Selective Electron Beam Melting) and LPBF printers known for accuracy and reliability, ensures that customers receive parts manufactured from well-characterized, high-quality feedstock, processed under stringent quality controls. Choosing a supplier with verifiable powder quality control and deep materials science knowledge is a non-negotiable aspect of sourcing mission-critical 3D printing aluminum comparison components like satellite brackets.
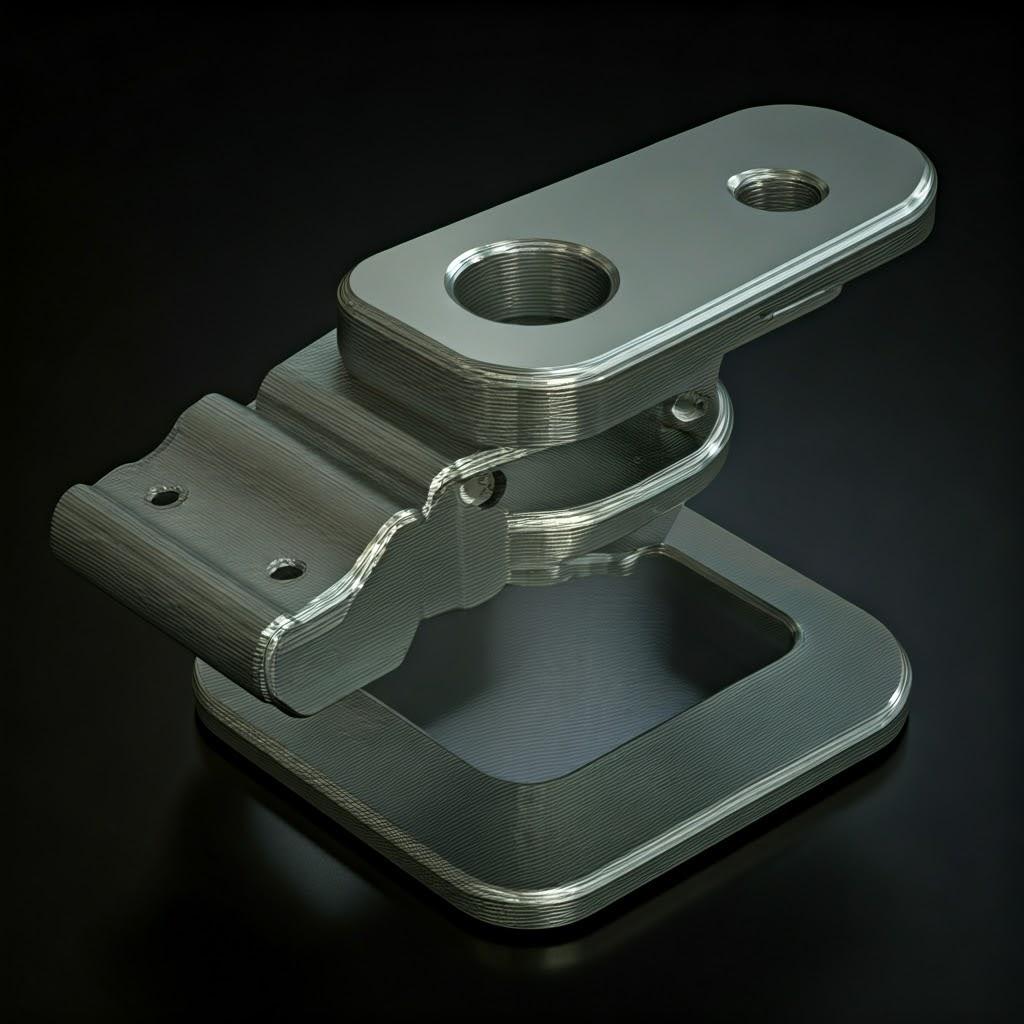
Design Considerations for Additive Manufacturing (DfAM) of Satellite Brackets
Successfully leveraging aluminum 3D printing for satellite brackets requires more than simply converting an existing traditionally manufactured design into a printable file. It demands a fundamental shift in design philosophy, embracing Design für additive Fertigung (DfAM) principles. DfAM is not just about ensuring a part dürfen be printed; it’s about actively utilizing the unique capabilities of additive processes like Laser Powder Bed Fusion (LPBF) to maximize performance, minimize weight, reduce cost, and integrate functionality in ways previously unattainable. For engineers designing critical satellite hardware, mastering DfAM is key to unlocking the full potential of aluminum AM.
1. Embracing Topology Optimization and Generative Design:
- Moving Beyond Conventional Shapes: Forget the constraints of billets, blocks, and tool access inherent in machining. AM allows for freeform, organic shapes dictated by physics and performance requirements, not manufacturing limitations.
- Topology Optimization Workflow: This powerful computational technique lies at the heart of lightweighting structural components like brackets. The typical workflow involves:
- Defining the Design Space: Identifying the maximum allowable volume the bracket can occupy.
- Specifying Loads and Constraints: Applying realistic load cases (static, dynamic, thermal) that the bracket will experience during launch and in-orbit operation. Defining boundary conditions (where it’s fixed) and keep-out zones (areas needed for mounting other components or access).
- Setting Objectives: Usually, the primary objective is to minimize mass while satisfying constraints on maximum stress and deflection (or maximize stiffness for a given mass).
- Running the Optimization: Using specialized Software zur Topologieoptimierung (e.g., Altair Inspire, nTopology, ANSYS Discovery, Siemens NX) to iteratively remove material from areas experiencing low stress, leaving material only where it’s structurally necessary to carry the loads.
- Interpreting and Reconstructing: The raw output is often a mesh representation that needs interpretation and conversion into smooth, manufacturable CAD geometry (often involving NURBS surfacing or implicit modeling techniques). This step requires engineering judgment to ensure the result is practical and meets all functional needs.
- Generative Design Exploration: Taking optimization a step further, generative design tools can autonomously explore thousands of potential design solutions based on the defined constraints and goals, often presenting non-intuitive but highly efficient structural concepts.
- Ergebnis: Brackets that look radically different from their machined counterparts – often resembling bone structures or complex trusses – but offering substantial weight savings (often 30-70%) while meeting or exceeding performance requirements.
2. Leveraging Lattice Structures:
- Engineered Micro-Architectures: AM uniquely enables the integration of complex internal lattice structure design AM within solid components. These repeating unit cells (e.g., cubic, diamond, octet-truss, gyroids, TPMS – Triply Periodic Minimal Surfaces) can be used strategically within a bracket design:
- Enhanced Stiffness-to-Weight: Filling certain volumes with low-density lattices instead of solid material can significantly increase overall stiffness with minimal weight gain.
- Schwingungsdämpfung: Specific lattice geometries can be designed to absorb or dissipate vibrational energy, crucial for protecting sensitive equipment mounted on the bracket.
- Wärmemanagement: Open-cell lattices allow for fluid flow (if needed for active cooling) or increased surface area for passive heat dissipation.
- Überlegungen zum Design: Requires careful selection of lattice type, cell size, strut/wall thickness, and smooth transitions to solid sections to avoid stress concentrations and ensure printability. Analysis tools are needed to predict the effective mechanical properties of the lattice region.
3. Adhering to LPBF Process Constraints (Aluminum Specific):
- Minimale Featuregröße: There’s a limit to how small features can be reliably printed. Minimum wall thickness is typically around 0.4-0.8 mm, varying with the machine and material. Minimum hole diameters are also constrained.
- Overhangs and Support Structures: LPBF builds parts layer by layer. Surfaces parallel to the build plate print well, as do vertical walls. However, angled surfaces (“overhangs”) require support structures beneath them once the angle drops below a certain threshold relative to the build plate (typically below 45 degrees for aluminum).
- Selbsttragende Winkel: Design components to maximize self-supporting angles (greater than 45 degrees) wherever possible to minimize the need for supports.
- Strategie unterstützen: Where supports are unavoidable, they must be designed carefully. They add print time, consume material, require post-processing for removal, and can affect the surface finish of the supported area. Design supports that are strong enough to prevent distortion but easy to remove without damaging the part (e.g., using reduced contact points, perforations). Avoid large, flat downward-facing surfaces (requiring extensive supports) close to the build plate.
- Reststress-Management: Incorporate design features that help mitigate residual stress build-up, such as rounded corners instead of sharp edges, and avoiding abrupt changes in cross-section. Build orientation also plays a critical role.
- Ausrichtung der Löcher: Horizontal holes often print with better circularity than vertical holes due to the layer-wise building, though vertical holes might have smoother internal surfaces. Consider orientation based on functional requirements.
- Teilentfernung: Design features or consider orientation to facilitate easier removal of the part from the build plate after printing (often requiring wire EDM or sawing).
4. Integrating Functionality:
- Beyond Structure: Think beyond just holding things in place. Can the bracket also serve other purposes?
- Cable/Wire Management: Integrate channels, clips, or routing paths directly into the bracket structure.
- Wärmemanagement: Design integrated heat sinks, channels for fluid cooling, or features that promote conductive heat transfer.
- Fluid Handling: For propulsion or thermal systems, internal channels for fluid flow can be incorporated, eliminating the need for separate tubing and fittings.
- Kinematic Features: Integrate hinges, pivots, or compliant mechanisms directly into the bracket design.
5. Simulation-Driven Design and Validation:
- Virtual Testing: Given the complex geometries often resulting from topology optimization and lattice structures, FEA simulation AM parts is absolutely critical. Perform structural analysis (static, dynamic, buckling) and thermal analysis on the proposed AM design vor committing to printing.
- Anisotropy Consideration: LPBF parts can exhibit some degree of anisotropy (different mechanical properties in different directions relative to the build layers). Advanced simulations may need to account for this.
- As-Printed Performance: Simulation should ideally predict the performance of the final, post-processed part, considering the effects of heat treatment and any machining operations. Comparing simulation results with physical test data from printed coupons is essential for validating models.
Partnering for DfAM Expertise:
Mastering DfAM requires experience. Engaging with AM service providers like Met3dp, who possess deep knowledge of specific Druckverfahren like LPBF and materials like AlSi10Mg and Scalmalloy®, can be invaluable. They often provide DfAM services aerospace customers rely on, offering guidance on optimizing designs for printability, performance, and cost-effectiveness, ensuring that the unique advantages of AM are fully realized for demanding satellite applications.
Achievable Tolerance, Surface Finish, and Dimensional Accuracy in Aluminum AM
While metal additive manufacturing offers unparalleled design freedom, it’s crucial for engineers and procurement managers to have realistic expectations regarding the achievable precision compared to traditional high-precision manufacturing methods like CNC machining. Understanding the typical tolerances, surface finish characteristics, and factors influencing dimensional accuracy in Laser Powder Bed Fusion (LPBF) of aluminum alloys is essential for designing functional satellite brackets and planning necessary post-processing steps.
1. Dimensional Tolerances:
- Allgemeine Erwartungen: As a general guideline, typical Toleranzen beim 3D-Druck von Metall for medium-sized aluminum parts produced via LPBF fall within the range of ±0.1 mm to ±0.2 mm for smaller features (up to ~50-100 mm), and ±0.1% to ±0.2% of the nominal dimension for larger features. For instance, a 200 mm long feature might have a tolerance of ±0.2 mm to ±0.4 mm.
- Comparison to Machining: These tolerances are generally wider than what can be achieved with precision CNC machining (which can often hold tolerances of ±0.01 mm to ±0.05 mm or tighter). Therefore, AM parts are often considered “near-net shape,” especially for critical interfaces.
- Faktoren, die die Verträglichkeit beeinflussen:
- Kalibrierung der Maschine: Regular calibration and maintenance of the LPBF system are critical.
- Prozessparameter: Laser power, scan speed, layer thickness, and hatching strategy affect melt pool stability and shrinkage.
- Thermische Effekte: Residual stresses built up during the rapid heating and cooling cycles can cause distortion and warping, affecting final dimensions, particularly in large or geometrically complex parts.
- Größe und Geometrie der Teile: Larger parts and those with significant unsupported overhangs or thin walls are more prone to deviation.
- Orientierung aufbauen: The orientation of the part on the build plate influences thermal history and support requirements, impacting dimensional accuracy.
- Nachbearbeiten: Stress relief heat treatments can cause minor dimensional changes. Support removal and subsequent machining operations obviously affect final dimensions.
2. Oberflächengüte (Rauhigkeit):
- As-Built Roughness: The surface finish of as-built LPBF aluminum parts is inherently rougher than machined surfaces. Typical average roughness (Ra) values range from 10 µm to 25 µm. This roughness comes from:
- Partially Melted Powder: Powder particles sintered to the surface.
- Layer Lines: Visible lines corresponding to the individual layers (typically 20-60 µm thick).
- Stair-Stepping Effect: On angled or curved surfaces, the layer-wise approximation creates a “stair-step” texture, which is more pronounced on surfaces with shallow angles relative to the build plate. Top-facing surfaces (parallel to the build plate) tend to be smoother than side walls or up/down-facing angled surfaces. Down-facing surfaces where supports were attached are often the roughest after support removal.
- Impact on Performance: This as-built LPBF surface finish aluminum may be acceptable for some non-critical surfaces, but it can negatively impact fatigue life (surface roughness acts as stress concentrators) and may not be suitable for sealing surfaces or interfaces requiring smooth contact.
- Verbesserung der Oberflächengüte: Post-processing steps like bead blasting, tumbling, chemical etching, or polishing are typically required to achieve smoother finishes (e.g., Ra < 5 µm or even < 1 µm with polishing).
3. Dimensional Accuracy and Geometric Control:
- Overall Form: Beyond feature tolerances, Maßhaltigkeit additive Fertigung also relates to the overall geometric fidelity of the part compared to the original CAD model (e.g., flatness, parallelism, circularity).
- Verformung und Verzerrung: As mentioned, thermal stresses are a major challenge. Careful process simulation, optimized part orientation, robust support structures, and controlled heating/cooling strategies are employed by experienced AM providers to minimize warping and ensure the part maintains its intended shape.
- Interne Merkmale: AM allows for complex internal channels, but verifying their geometry and ensuring they are free from obstructions (like trapped powder) requires specialized inspection.
4. Metrology and Inspection for Aerospace Qualification:
Given the critical nature of satellite components, rigorous inspection and metrology are non-negotiable. Standard measurement techniques are adapted and augmented for AM parts:
- Koordinatenmessmaschinen (KMG): Used for high-precision measurement of specific features, datums, and critical dimensions, particularly after final machining operations. CMM-Prüfung Luft- und Raumfahrt Die Protokolle sind gut etabliert.
- 3D-Scannen: Techniques like structured light or laser scanning capture millions of points on the part’s surface, creating a detailed 3D model that can be compared directly to the original CAD data. This is excellent for verifying overall shape, identifying unexpected deviations, and performing 3D scanning quality control.
- Computertomographie (CT) Scannen: This X-ray based technique is invaluable for non-destructively inspecting the internal structure of AM parts. CT scanning AM parts allows for:
- Porosity Detection: Identifying the size, location, and distribution of internal voids (gas or lack-of-fusion porosity) which can affect mechanical properties.
- Internal Geometry Verification: Measuring the dimensions and confirming the clearance of internal channels or complex features.
- Overall Density Measurement: Assessing the consistency of the material consolidation.
- Materialprüfung: Destructive testing of witness coupons printed alongside the actual parts is crucial for verifying that the material meets the required mechanical properties (tensile strength, yield strength, elongation, fatigue life).
In summary, while aluminum AM via LPBF produces near-net shape parts with remarkable geometric freedom, engineers must design with achievable tolerances and surface finishes in mind. Critical dimensions and surfaces will almost always require post-process machining. Robust metrology and inspection plans, often incorporating CMM, 3D scanning, and CT scanning, are essential for qualifying 3D printed aluminum brackets for demanding aerospace part inspection Normen.
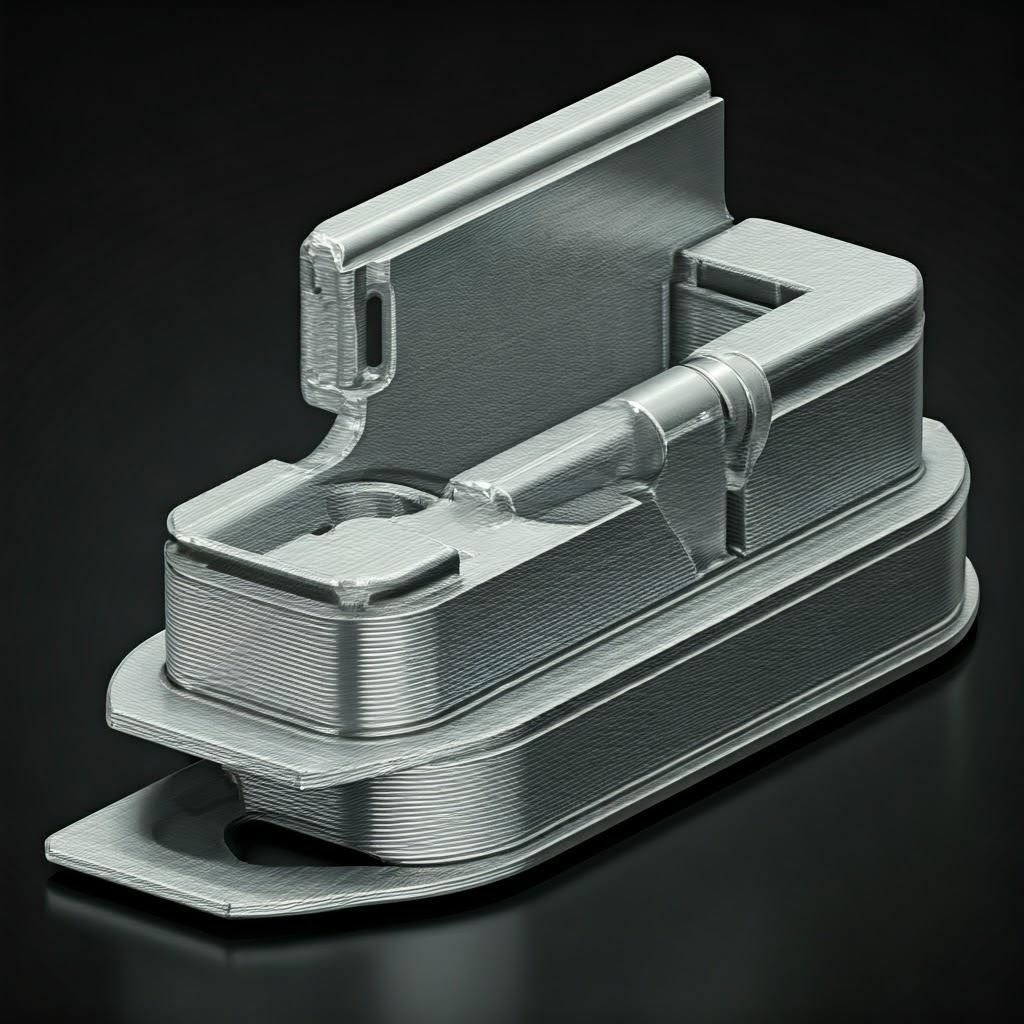
Post-Processing Requirements for Mission-Critical Satellite Brackets
Printing an aluminum satellite bracket using Laser Powder Bed Fusion (LPBF) is often just the first manufacturing step. To transform the raw, as-built part into a functional, flight-ready component, a series of crucial post-processing steps are typically required. These steps are essential for relieving internal stresses, achieving the desired mechanical properties, removing support structures, attaining the required surface finish and dimensional tolerances, and ensuring the cleanliness demanded by the space environment. Understanding these requirements is vital for planning production timelines and costs.
1. Stress Relief and Heat Treatment:
- Why it’s Necessary: The rapid heating and cooling inherent in the LPBF process create significant thermal gradients within the part as it’s built layer by layer. This leads to the build-up of internal residual stresses. These stresses can cause distortion (warping) during or after printing, cracking, and can negatively impact the part’s mechanical performance and fatigue life.
- Stressabbau: Often the first step after printing (sometimes performed while the part is still attached to the build plate) is a stress relief heat treatment. This involves heating the part to a specific temperature (below the aging temperature for AlSi10Mg) and holding it for a period, followed by slow cooling. This reduces the internal stresses without significantly altering the microstructure or hardness.
- Solution Annealing and Aging (e.g., T6 for AlSi10Mg): For alloys like AlSi10Mg, a full Wärmebehandlung AlSi10Mg T6 cycle is commonly applied to achieve optimal mechanical properties (strength and hardness). This involves:
- Lösungsglühen: Heating to a high temperature (e.g., ~530°C) to dissolve alloying elements (Si, Mg) into the aluminum matrix.
- Abschrecken: Rapidly cooling (usually in water or polymer) to trap these elements in a supersaturated solid solution.
- Künstliche Alterung: Reheating to a lower temperature (e.g., ~160-170°C) for several hours, causing fine precipitates to form within the aluminum matrix, which significantly increases strength and hardness.
- Scalmalloy® Heat Treatment: Scalmalloy® typically achieves its high strength in the as-built or stress-relieved state due to its unique precipitation behavior during the printing process itself. Specific stress relief cycles might be recommended by the material supplier depending on the application’s requirements.
- Atmosphärenkontrolle: Heat treatments are usually performed in controlled atmospheres (vacuum or inert gas) to prevent oxidation, especially at high temperatures.
2. Build Plate Removal and Support Structure Removal:
- Separation: The part(s) must be carefully removed from the build plate. This is often done using wire Electrical Discharge Machining (EDM), sawing, or CNC milling.
- Support Removal Techniques: Removing the support structures generated during printing requires careful manual or automated work. Common methods include:
- Manual Breaking/Clipping: For supports designed with weak interfaces.
- Hand Grinding/Filing: To remove remaining support nubs.
- CNC-Bearbeitung: For precise removal or accessing difficult areas.
- Drahterodieren: Can sometimes be used for intricate support removal.
- Herausforderungen: AM support removal can be labor-intensive and risks damaging the part’s surface if not done carefully. Design for Additive Manufacturing (DfAM) plays a crucial role in minimizing the need for supports and designing them for easier removal. Residual marks (“witness marks”) are often left on the surfaces where supports were attached, which may require further finishing.
3. Surface Finishing:
The as-built surface of LPBF parts is generally too rough for many aerospace applications. Various finishing steps can be applied:
- Perlstrahlen / Shot Peening: Propelling small beads (glass, ceramic) or shots (metal) at the surface. Bead blasting aluminum creates a uniform, non-directional matte finish, removes loose powder particles, and can impart beneficial compressive residual stresses (shot peening) to improve fatigue life.
- Tumbling / Vibro-Finishing: Placing parts in a tumbler or vibratory bowl with abrasive media to smooth surfaces and round sharp edges. Effective for batches of smaller parts.
- Polieren: Manual or automated polishing can achieve very smooth, mirror-like finishes (Ra < 0.1 µm) on specific surfaces where required (e.g., sealing faces, optical mounts), but it is labor-intensive.
- Eloxieren: An electrochemical process that creates a hard, durable aluminum oxide layer on the surface. Anodizing 3D printed parts (AlSi10Mg and Scalmalloy® can both be anodized) enhances corrosion resistance, improves wear resistance, provides electrical insulation, and allows for coloring (though functional coatings are usually preferred over decorative ones in space). Different types exist (e.g., Type II sulfuric, Type III hardcoat).
- Chemical Conversion Coating (e.g., Alodine® / Chromate / Non-Chromate): Creates a thin chemical film that improves corrosion resistance and provides an excellent adhesion base for paints or coatings. Essential for aerospace surface treatment compliance. Specific types (e.g., MIL-DTL-5541 compliant) are often required for space applications.
4. Precision CNC Machining:
As LPBF tolerances may not meet the requirements for all features, CNC machining AM components is a common and often necessary post-processing step. This hybrid manufacturing approach leverages the geometric freedom of AM and the precision of CNC:
- Critical Interfaces: Machining mating surfaces, mounting holes, bearing seats, and other features requiring tight tolerances (typically tighter than ±0.1 mm).
- Versiegeln von Oberflächen: Achieving the flatness and smoothness required for O-ring grooves or other sealing applications.
- Fäden: Creating precise threaded holes or features.
- Verbesserung der Oberflächengüte: Machining specific surfaces to achieve a defined roughness value.
- Workflow: The near-net shape part is printed via LPBF, heat treated, and then transferred to a CNC machine where datums are established, and critical features are machined to final specifications.
5. Cleaning and Passivation:
- Stringent Requirements: Satellite component cleaning protocols are extremely rigorous. Any residual contaminants (powder particles, machining fluids, fingerprints, organic residues) can cause problems in the vacuum and thermal environment of space (e.g., outgassing, corrosion, electrical issues).
- Reinigungsverfahren: Multi-stage cleaning processes involving ultrasonic baths, specific solvents, detergents, and high-purity water rinses are typically employed. Procedures must be carefully documented and validated.
- Passivierung: While often associated with stainless steel, specific passivation treatments might be applied to aluminum alloys to ensure a stable, inert surface oxide layer.
Each of these post-processing steps adds time and cost to the production of a 3D printed satellite bracket. It is crucial to consider these requirements early in the design phase and work with an AM provider who has validated processes and expertise in performing these operations to aerospace standards.
Common Challenges in 3D Printing Aluminum Brackets and Mitigation Strategies
While aluminum LPBF offers transformative potential for satellite brackets, the process is not without its challenges. Achieving the consistent quality, reliability, and performance demanded by the aerospace industry requires careful control, deep process understanding, and proactive mitigation strategies. Awareness of these potential pitfalls is essential for both designers and procurement teams when specifying and sourcing AM components.
1. Eigenspannung, Verformung und Verziehen:
- Die Herausforderung: The intense, localized heating by the laser and subsequent rapid cooling create steep thermal gradients within the part and between the part and the build plate. This leads to differential expansion and contraction, resulting in significant internal residual stresses. If these stresses exceed the material’s yield strength at elevated temperatures, they can cause distortion (deviation from the intended shape), warping (especially lifting of edges/corners), or even cracking during the build or upon removal from the build plate. Metal AM residual stress ist ein Hauptanliegen.
- Strategien zur Schadensbegrenzung:
- Prozess-Simulation: Utilizing software to simulate the build process, predict thermal gradients and stress accumulation, and identify potential problem areas before printing.
- Optimierte Gebäudeausrichtung: Orienting the part on the build plate to minimize large flat areas parallel to the plate, reduce overhangs, and manage heat distribution.
- Robuste Stützstrukturen: Designing supports not just to hold overhangs but also to anchor the part firmly to the build plate, resisting warping forces and acting as heat sinks.
- Optimierte Scan-Strategie: Using specific laser scanning patterns (e.g., island scanning, chessboard patterns) to manage heat input and reduce localized stress peaks.
- Build Plate Heating: Preheating the build plate reduces the thermal gradient between the printed part and the plate.
- Stressabbau nach der Bauphase: Applying appropriate heat treatment immediately after the build (often before removing supports) is crucial to relax residual stresses.
2. Porosity Control:
- Die Herausforderung: Porosity refers to small voids within the printed material. Common types in LPBF include:
- Gas Porosität: Caused by dissolved gases (often Hydrogen in aluminum) trapped in the melt pool as it solidifies. Can also result from gas bubbles within the atomized powder particles.
- Lack-of-Fusion (LoF) Porosity: Irregularly shaped voids occurring between layers or scan tracks due to insufficient melting/fusion, often caused by incorrect process parameters (e.g., too low laser power, too high scan speed).
- Auswirkungen: Porosity acts as stress concentrators, significantly reducing ductility, fatigue life, and fracture toughness – properties critical for aerospace components. LPBF porosity defects must be minimized.
- Strategien zur Schadensbegrenzung:
- Hochwertiges Pulver: Using powder with high sphericity, low internal porosity, controlled particle size distribution, and low moisture/dissolved gas content. This starts with advanced powder manufacturing techniques, like those employed by Met3dp for its Metallpulver. Proper storage and handling under inert conditions are vital.
- Optimierte Prozessparameter: Developing and meticulously controlling laser power, scan speed, layer thickness, hatch spacing, and inert gas atmosphere (high-purity Argon flow to remove fumes and prevent oxidation) to ensure complete melting and fusion. Parameter sets are often specific to the machine and material batch.
- Wartung der Maschine: Ensuring the laser system, optics, and gas flow systems are clean and functioning correctly.
- Zerstörungsfreie Prüfung (NDT): Using CT scanning to detect, quantify, and characterize internal porosity in finished parts or test coupons. Establishing clear acceptance criteria based on porosity levels.
3. Support Removal Difficulties:
- Die Herausforderung: While essential, support structures can be difficult and time-consuming to remove, especially from complex internal geometries or delicate features. Improper removal can damage the part surface or leave residual stresses. Designing supports involves a trade-off: they must be strong enough to function during the build but easy enough to remove afterward.
- Strategien zur Schadensbegrenzung:
- DfAM for Support Reduction: Designing parts with self-supporting angles (>45°) wherever possible, using chamfers instead of small overhangs, and orienting the part strategically to minimize supported areas.
- Optimiertes Support-Design: Using specialized support structure optimization techniques (e.g., tree supports, block supports with minimal contact points or perforations, conical supports) tailored to the geometry and material.
- Process Parameters for Supports: Using different laser parameters for support structures to make them less dense or more brittle for easier removal.
- Nachbearbeitungsplanung: Selecting appropriate removal methods (manual, CNC, EDM) based on the support type and location. Budgeting sufficient time and resources for careful removal.
4. Powder Handling, Quality Control, and Traceability:
- Die Herausforderung: Ensuring the quality and consistency of the aluminum powder throughout its lifecycle (from production to storage, handling, printing, and recycling) is critical for aerospace powder management. Contamination (e.g., with other metals, oxides, organics) or changes in particle size distribution can degrade part quality and mechanical properties. Full traceability of powder batches used for specific flight hardware is mandatory.
- Strategien zur Schadensbegrenzung:
- Supplier Qualification: Sourcing powder from reputable suppliers like Met3dp with robust quality control and batch testing.
- Strict Handling Protocols: Implementing dedicated equipment, controlled environments (humidity control, inert atmosphere), and documented procedures for powder loading, unloading, sieving, and storage.
- Pulver-Recycling-Strategie: Implementing validated procedures for recycling unused powder, including tracking the number of reuse cycles and periodic testing to ensure quality is maintained. Blending virgin and recycled powder according to qualified ratios.
- Rückverfolgbarkeit der Chargen: Maintaining meticulous records linking specific powder batches (and reuse history) to the parts printed with them (required for AM quality assurance aerospace).
5. Ensuring Consistent Mechanical Properties:
- Die Herausforderung: Achieving repeatable mechanical properties (strength, ductility, fatigue life) from build-to-build, between different machines, and even within different locations on the same build plate can be challenging due to the process’s sensitivity to numerous variables. Parts can also exhibit anisotropy (properties varying with direction relative to build layers).
- Strategien zur Schadensbegrenzung:
- Process Standardization and Control: Implementing rigorous process monitoring, maintaining tight control over all critical parameters, and using standardized, validated post-processing procedures (especially heat treatment).
- Machine Calibration and Maintenance: Regular calibration of laser power, scanner accuracy, and environmental controls.
- Prüfung der Materialeigenschaften: Printing and testing witness coupons alongside actual parts in every build according to aerospace standards (e.g., ASTM, MMPDS) to verify properties achieve minimum specifications. Testing coupons in different orientations (X, Y, Z) to characterize anisotropy.
- Statistische Prozesskontrolle (SPC): Monitoring key process indicators and material properties over time to ensure stability and identify potential deviations.
- Partnership with Experienced Providers: Collaborating with AM service providers like Met3dp who possess mature processes, robust quality management systems (QMS), and a proven track record in delivering consistent AM part properties for critical applications. Their experience helps navigate and mitigate these inherent challenges effectively.
Addressing these challenges requires a combination of advanced technology, meticulous process control, robust quality systems, and deep engineering expertise. By understanding these potential issues and working with qualified partners, the aerospace industry can confidently adopt aluminum AM for lightweight, high-performance satellite brackets.
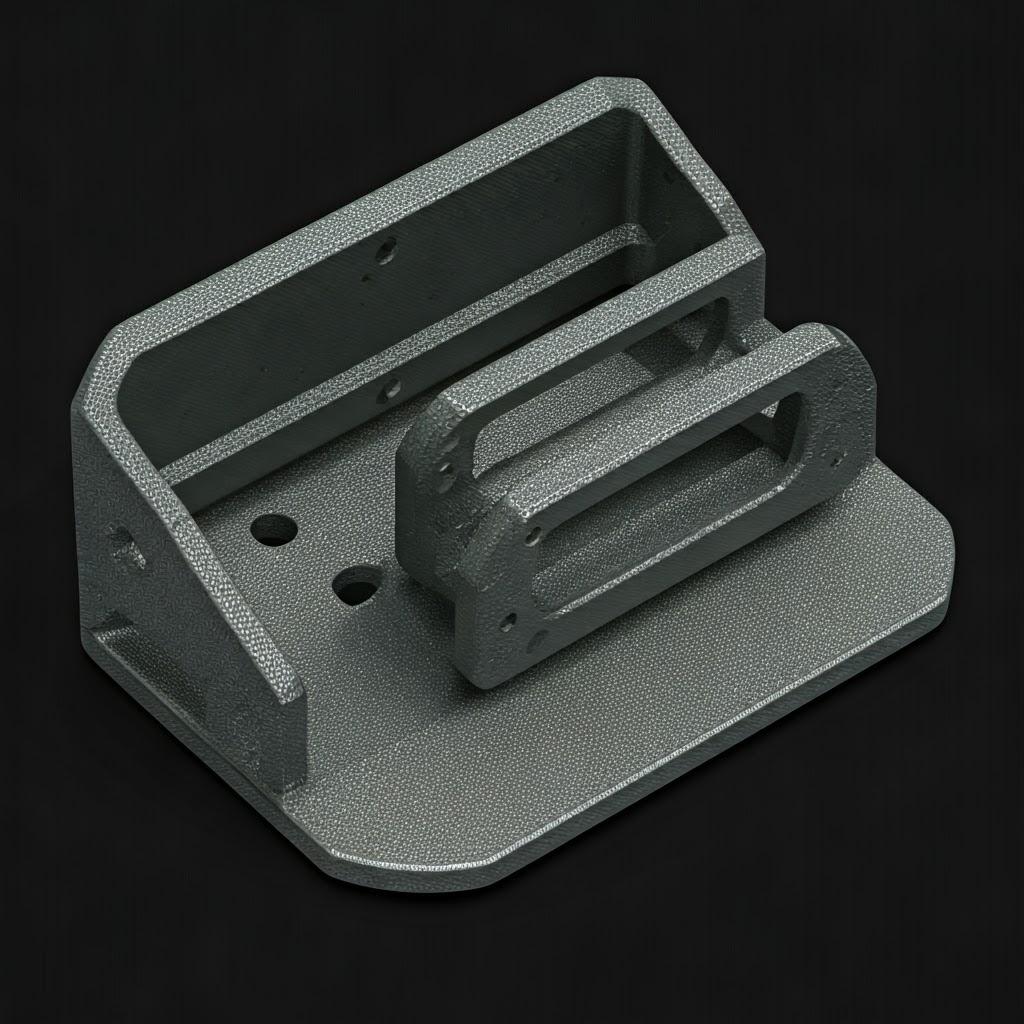
How to Choose the Right Metal 3D Printing Service Provider for Satellite Components
Selecting the right manufacturing partner is as critical as the design and material choice when producing mission-critical hardware like satellite brackets using additive manufacturing. The unique demands of the aerospace industry – stringent quality requirements, complex geometries, advanced materials, and the need for absolute reliability – necessitate partnering with a metal AM service provider that possesses specialized expertise, robust processes, and the right certifications. Making the wrong choice can lead to project delays, cost overruns, subpar part quality, and potentially catastrophic component failure. For procurement managers and engineering teams, a thorough evaluation process is essential. Here are key criteria to consider when conducting aerospace AM supplier qualification:
1. Essential Certifications and Quality Management System (QMS):
- AS9100-Zertifizierung: This is the internationally recognized Quality Management System standard for the Aviation, Space, and Defense (ASD) industry. AS9100 incorporates ISO 9001 requirements but adds stringent controls specific to aerospace, covering areas like configuration management, risk management, traceability, supplier control, and prevention of counterfeit parts. Partnering with an AS9100 certified 3D printing provider (or one actively demonstrating compliance and pursuing certification) provides significant assurance that rigorous quality processes are in place.
- Zertifizierung nach ISO 9001: The foundational QMS standard, indicating that the provider has established and maintains processes for consistent quality, customer satisfaction, and continuous improvement. While essential, AS9100 is the preferred standard for aerospace work.
- Robust QMS Implementation: Beyond the certificate, evaluate the implementation of their QMS. Does it specifically address the nuances of additive manufacturing? Key aspects include:
- Prozess-Validierung: Documented evidence that their LPBF processes for specific materials (AlSi10Mg, Scalmalloy®) consistently produce parts meeting predefined specifications (e.g., density, mechanical properties).
- Prozessbegleitende Überwachung: Systems for monitoring critical process parameters during the build (e.g., laser power, oxygen levels, melt pool characteristics).
- Zerstörungsfreie Prüfung (NDT): In-house or qualified third-party capabilities for CT scanning, ultrasonic testing, or other methods relevant to AM part inspection.
- Metrology Capabilities: Calibrated CMMs, 3D scanners, and other metrology equipment suitable for verifying AM part dimensions and geometry.
- Documentation Control: Rigorous procedures for managing design data, process parameters, inspection results, and material traceability records.
- Personnel Training: Ensuring operators, engineers, and inspectors are adequately trained and qualified for their roles in the AM workflow. An effective additive manufacturing QMS is comprehensive and deeply integrated.
2. Proven Aerospace Experience and Material Expertise:
- Erfolgsbilanz: Has the provider successfully manufactured components for aerospace or satellite applications before? Can they provide relevant case studies, references, or examples (while respecting confidentiality)? Experience with the specific challenges of space hardware (e.g., outgassing requirements, thermal cycling, radiation effects) is invaluable.
- Material-Spezialisierung: Do they have deep expertise in processing the specific aluminum alloys you require (AlSi10Mg, Scalmalloy®)? This includes validated parameter sets, understanding of microstructure-property relationships, and proven post-processing protocols (especially heat treatments). Ask about their experience qualifying these materials for demanding applications.
- Powder Management Expertise: Scrutinize their procedures for aerospace powder management:
- Powder sourcing and supplier qualification.
- Incoming powder inspection and testing.
- Controlled storage environment (humidity, temperature).
- Handling procedures to prevent contamination and ensure operator safety.
- Powder traceability (batch tracking).
- Validated powder recycling/reuse strategy and tracking. Providers like Met3dp, who also manufacture high-quality powders using advanced methods like gas atomization, often have a distinct advantage here.
3. Equipment, Technology, and Facility:
- Industrial-Grade Machines: Are they using reputable, industrial-grade LPBF equipment capabilities known for stability, reliability, and consistency? Ask about their specific machine models and configurations.
- Machine Maintenance and Calibration: Do they have documented procedures and records for regular machine maintenance, calibration (laser power, scanner accuracy), and system health monitoring?
- Environmental Controls: Is the facility environmentally controlled (temperature, humidity) to ensure process stability and powder integrity?
- Kapazität und Redundanz: Do they have sufficient machine capacity to meet your project’s potential volume and lead time requirements? Is there redundancy in case of machine downtime?
4. Engineering Support and DfAM Services:
- Fachwissen im Bereich Design für additive Fertigung (DfAM): Bietet der Anbieter DfAM services aerospace customers need? Can their engineers review your designs and provide constructive feedback to optimize for printability, reduce support structures, enhance performance, or lower cost? This collaborative approach is often crucial for maximizing AM’s benefits.
- Build Simulation Capabilities: Can they perform build process simulations to predict potential issues like distortion or stress concentration and optimize the build setup (orientation, supports)?
- Problemlösung: Do they have experienced engineers who can help troubleshoot issues that may arise during design, printing, or post-processing?
5. Communication, Lead Times, and Total Cost:
- Responsiveness and Communication: How responsive are they to inquiries and technical questions? Is there a clear point of contact? Good communication is vital throughout the project lifecycle.
- Quoting Process: Is their quoting process transparent? Does it clearly break down costs associated with material, printing, post-processing, and QA?
- Lead Time Realism: Can they provide realistic lead time estimates based on their current workload and the project’s complexity? Do they have a track record of meeting deadlines?
- Total Value Proposition: Don’t choose solely based on the lowest price per part. Consider the “total cost” which includes the value of their expertise, the robustness of their quality system, the level of engineering support, and the mitigation of risks associated with producing critical flight hardware. A slightly higher price from a highly qualified provider often represents better overall value and lower project risk. Evaluate potential partners based on their ability to form a true metal AM partnership.
Evaluating Potential Partners like Met3dp:
When evaluating potential suppliers, consider companies like Met3dp. Headquartered in Qingdao, China, Met3dp positions itself as a leading provider of comprehensive additive manufacturing solutions, specializing in both 3D printing equipment (including SEBM and LPBF systems known for industry-leading volume, accuracy, and reliability) and the high-performance metal powders crucial for industrial applications. Their use of advanced powder manufacturing techniques (Gas Atomization, PREP) and their portfolio encompassing not just aluminum alloys but also titanium alloys (TiNi, TiTa, TiAl, TiNbZr), CoCrMo, stainless steels, and superalloys indicate deep materials science expertise. Their focus on mission-critical parts for aerospace, medical, and automotive fields suggests experience with demanding quality requirements. While specific certifications like AS9100 should always be verified directly, their positioning as a comprehensive solution provider offering application development services implies a strong engineering support capability. A thorough evaluation against the criteria listed above would determine their suitability for a specific satellite bracket project.
Die Wahl des richtigen metal AM service bureau selection requires due diligence. Request facility tours (virtual or in-person), audit their QMS documentation, review case studies, and engage in detailed technical discussions to ensure they have the capabilities, expertise, and quality commitment necessary for your critical satellite components.
Cost Factors and Lead Time for 3D Printed Aluminum Satellite Brackets
Understanding the financial and temporal investments required for producing aluminum satellite brackets via LPBF is crucial for project planning, budgeting, and comparing AM against alternative manufacturing methods. Both cost and lead time are influenced by a complex interplay of factors related to design, material, processing, and quality requirements.
Breakdown of Cost Drivers:
Die Kostenanalyse für den 3D-Druck von Metall for a satellite bracket typically involves several key components:
- 1. Material Costs:
- Pulvertyp: The base cost of the metal powder. High-performance alloys like Scalmalloy® are significantly more expensive than standard AlSi10Mg due to alloying elements (Scandium) and licensing/development costs.
- Teilband: The amount of powder directly consumed to build the actual bracket. Larger or denser parts naturally cost more.
- Unterstützungsstruktur Volumen: Powder used to build the necessary support structures, which is later removed and often only partially recyclable. Optimized designs minimize support volume.
- Powder Handling & Waste: Costs associated with powder management, sieving, quality testing, and unavoidable minor losses during handling.
- 2. Machine Time Costs:
- Vorbereitung des Baus: Labor and time involved in setting up the build file (orientation, supports, slicing, parameter assignment) and preparing the machine.
- Druckzeit: The time the LPBF machine is actively running to print the part(s). This is heavily influenced by:
- Part Height: Primarily dictates print time, as each layer takes a certain amount of time to deposit and fuse, regardless of the layer’s surface area (within limits).
- Teil Volumen/Komplexität: Affects the amount of laser scanning required per layer.
- Schichtdicke: Thinner layers provide better resolution but significantly increase print time.
- Scan-Strategie: Complex hatching patterns or specific parameter requirements can influence speed.
- Verschachtelung: Printing multiple parts (identical or different) within a single build job can significantly reduce the per-part machine time cost by optimizing build plate utilization.
- Machine Operation & Depreciation: Hourly rates factor in machine cost, maintenance, consumables (filters, inert gas), energy, and facility overheads.
- 3. Post-Processing Costs:
- Arbeitsintensität: Many post-processing steps are labor-intensive (e.g., manual support removal, polishing, detailed inspection).
- Wärmebehandlung: Costs associated with furnace time, energy, controlled atmosphere, and labor for stress relief and/or T6 cycles.
- Unterstützung bei der Entfernung: Labor and potentially specialized equipment (EDM, CNC) costs.
- Bearbeitungen: Costs for CNC machine time, tooling, programming, and skilled labor, particularly if complex multi-axis machining is needed for critical features.
- Oberflächenveredelung: Costs vary significantly depending on the method (bead blasting is relatively cheap; extensive polishing or specialized coating like aerospace-grade anodizing is more expensive).
- Reinigung: Costs for specialized equipment (ultrasonic cleaners), consumables (solvents, detergents), and labor for multi-stage aerospace cleaning protocols.
- 4. Quality Assurance and Inspection Costs:
- Level of Scrutiny: The more critical the bracket, the more extensive (and costly) the QA requirements.
- Inspection Methods: Costs associated with CMM programming and operation, 3D scanning and analysis, CT scanning (often charged per part or per hour), and destructive material testing (tensile tests, fatigue tests on witness coupons).
- Dokumentation: Labor involved in preparing comprehensive documentation packages (Certificates of Conformance, material certs, inspection reports, test data, traceability records).
- 5. Engineering and Design Costs:
- DfAM und Optimierung: Costs associated with engineering time for topology optimization, generative design, DfAM reviews, and build simulation, if performed by the service provider.
Cost Comparison with CNC Machining:
- Complexity Advantage (AM): For highly complex, topology-optimized brackets, AM can be significantly cheaper than trying to machine the same geometry from a solid block (which might be impossible or require extensive multi-axis machining and setups).
- Volume Disadvantage (AM): For simpler bracket designs produced in higher volumes, traditional CNC machining often becomes more cost-effective due to faster cycle times per part once set up.
- Tooling (AM Advantage): AM avoids the high upfront tooling costs associated with casting or molding.
- Buy-to-Fly Ratio (AM Advantage): AM typically uses material more efficiently than subtractive machining, reducing raw material costs, especially for complex parts requiring large initial billets for CNC.
- Total Cost of Ownership: Bei der Evaluierung additive manufacturing pricing aerospace, consider the downstream benefits of AM’s weight savings (reduced launch costs) which can often outweigh a higher per-part manufacturing cost.
Faktoren, die die Vorlaufzeit beeinflussen:
Die Schätzung der AM-Vorlaufzeit depends on numerous sequential and parallel activities:
- Vorverarbeitung: Design finalization, DfAM review, simulation (if needed), quote approval, build file preparation, scheduling/queuing for machine availability (can be significant depending on provider workload). (Days to Weeks)
- Drucken: Actual build time on the LPBF machine. (Hours to Multiple Days)
- Cool-Down: Allowing the build plate and parts to cool sufficiently before handling. (Hours)
- De-Powdering & Build Plate Removal: Removing excess powder and separating parts from the plate. (Hours)
- Nachbearbeiten:
- Stress Relief / Heat Treatment: Furnace cycle time (Hours to Days) + cooling.
- Support Removal: (Hours to Days, depending on complexity and method).
- CNC Machining: (Hours to Days, depending on complexity and setup).
- Surface Finishing: (Hours to Days, depending on method).
- Cleaning: (Hours).
- Inspection & Quality Assurance: CMM, CT scanning, documentation preparation. (Days)
- Versand: Logistics. (Days)
Typical Timelines & Optimization:
- Prototypen: For simpler brackets with minimal post-processing, prototypes might be delivered in 1-3 weeks.
- Produktionsteile: For flight-qualified brackets requiring full post-processing and rigorous QA, lead times of 3-8 weeks or longer are common.
- Optimization: Clearly defining requirements upfront, optimizing the design for manufacturability (DfAM for support reduction), efficient nesting of parts in a build, and clear communication with the provider can help streamline the process and potentially shorten lead times. Requesting a detailed schedule with your metal AM quote request is advisable.
Ultimately, both cost and lead time are highly project-specific. Obtaining detailed quotes from qualified providers based on finalized designs and clearly defined requirements is essential for accurate budgeting and scheduling.
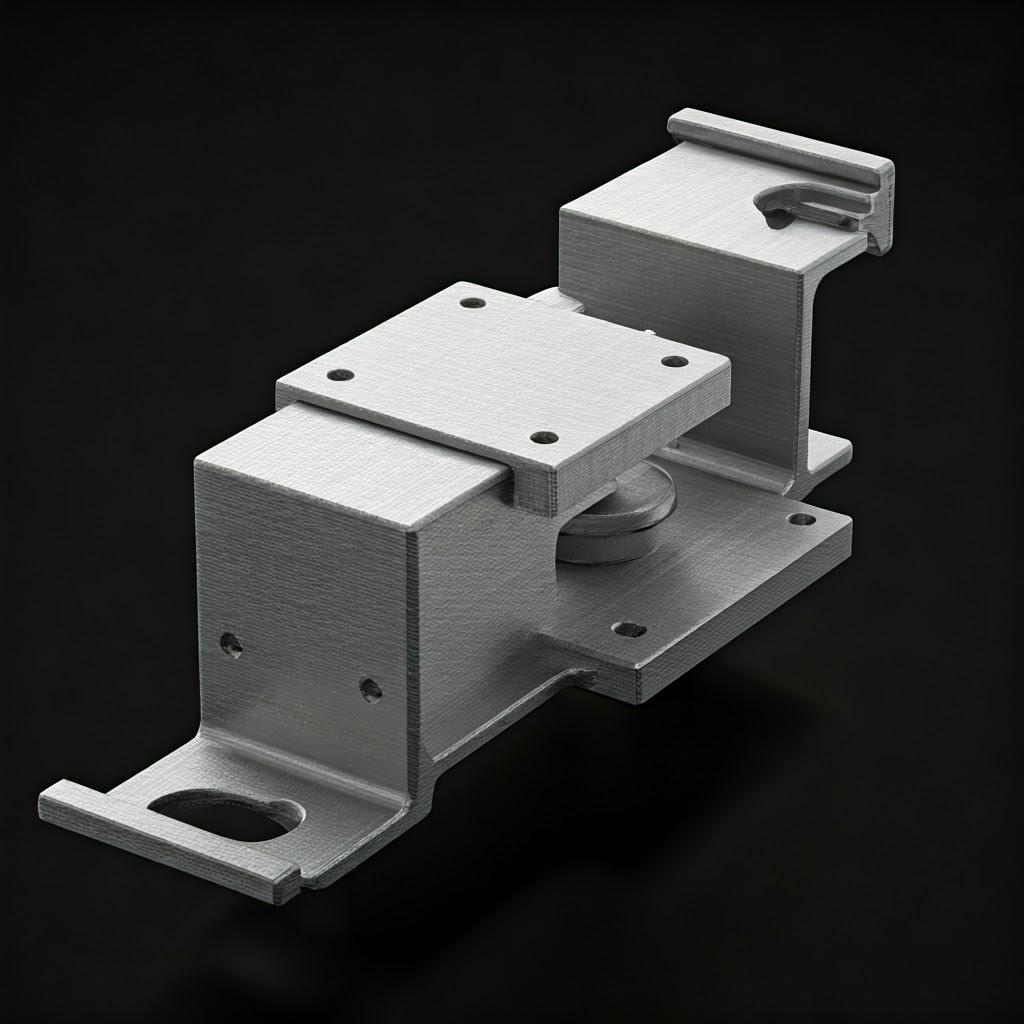
Frequently Asked Questions (FAQ) about Aluminum 3D Printed Satellite Brackets
Here are answers to some common questions engineers and procurement managers have when considering aluminum additive manufacturing for satellite brackets:
- Q1: What are the typical strength and stiffness values achievable with 3D printed AlSi10Mg and Scalmalloy®?
- A1: Properties depend heavily on process parameters and post-processing, but typical values are:
- AlSi10Mg (T6 Heat Treated): Yield Strength ≈ 230-300 MPa, Ultimate Tensile Strength ≈ 330-430 MPa, Elastic Modulus ≈ 70-75 GPa. Offers a good balance of properties suitable for moderately loaded parts.
- Scalmalloy® (As-Built/Stress Relieved): Yield Strength ≈ 450-520 MPa, Ultimate Tensile Strength ≈ 500-580 MPa, Elastic Modulus ≈ 70-76 GPa. Offers significantly higher 3D printed aluminum strength, approaching some titanium grades, ideal for high-stress, weight-critical applications. Stiffness (Modulus) is similar to AlSi10Mg. Always consult specific material datasheets from the provider for guaranteed minimums.
- A1: Properties depend heavily on process parameters and post-processing, but typical values are:
- Q2: How does the fatigue life of 3D printed aluminum compare to wrought aluminum or traditional designs?
- A2: This is complex. As-built LPBF parts often have lower fatigue life than wrought counterparts due to surface roughness and potential internal micro-defects (porosity). However:
- Scalmalloy® was specifically designed for high fatigue life Scalmalloy performance and generally outperforms AlSi10Mg and even some wrought alloys in fatigue tests, especially after appropriate surface treatments (like shot peening or polishing).
- AlSi10Mg fatigue life can be significantly improved with T6 heat treatment, Hot Isostatic Pressing (HIP – reduces porosity), and surface finishing, but may still be lower than high-strength wrought alloys.
- Design Matters: Topology optimized AM designs often reduce peak stresses compared to traditional blocky designs, which can improve the overall fatigue performance of the component, even if the base material fatigue strength is slightly lower. Careful design, material selection, process control, and post-processing are critical for fatigue-sensitive applications.
- A2: This is complex. As-built LPBF parts often have lower fatigue life than wrought counterparts due to surface roughness and potential internal micro-defects (porosity). However:
- Q3: Can complex internal channels for cooling or wiring be integrated into 3D printed brackets?
- A3: Yes, integrating AM internal channels is one of the key advantages of additive manufacturing. LPBF can create intricate, conformal cooling channels or pathways for routing small cables directly within the bracket structure, which would be impossible or extremely difficult with traditional methods. However, design considerations are crucial:
- Channels must be self-supporting or designed so internal supports can be removed (often challenging/impossible for very complex paths).
- Minimum channel diameter is limited by the process resolution and powder removal capabilities (typically > 1-2 mm).
- Thorough cleaning protocols are essential to ensure all residual powder is removed from internal channels, often verified using CT scanning or flow tests.
- A3: Yes, integrating AM internal channels is one of the key advantages of additive manufacturing. LPBF can create intricate, conformal cooling channels or pathways for routing small cables directly within the bracket structure, which would be impossible or extremely difficult with traditional methods. However, design considerations are crucial:
- Q4: What level of quality control and documentation is typically provided for aerospace-grade AM parts?
- A4: For flight hardware, comprehensive aerospace quality documentation AM is standard. A typical documentation package includes:
- Certificate of Conformance (CoC): Stating the parts meet the specified drawing requirements and standards.
- Materialzertifizierung: Traceability to the specific powder batch used, including supplier certificates verifying chemical composition and particle size distribution.
- Build Log: Record of the specific build job, machine used, key process parameters monitored during the build.
- Post-Processing Records: Confirmation and details of heat treatment cycles, machining operations, surface treatments performed.
- Inspektionsberichte: Dimensional inspection results (CMM report, 3D scan comparison), NDT reports (e.g., CT scan summary confirming density/lack of critical defects), and results of any visual inspections.
- Mechanical Test Results: Data from tensile tests, fatigue tests (if required), or hardness tests performed on witness coupons printed alongside the parts. The exact requirements are defined by the customer’s specifications and quality standards (like AS9100).
- A4: For flight hardware, comprehensive aerospace quality documentation AM is standard. A typical documentation package includes:
- Q5: What is the typical turnaround time for a prototype aluminum satellite bracket?
- A5: Satellite component prototyping time using LPBF is significantly faster than traditional methods involving tooling. For a typical prototype bracket requiring minimal post-processing (e.g., stress relief, basic support removal, bead blast), turnaround times can range from 1 to 3 weeks, depending on part complexity, provider workload/queue, and machine availability. If specific heat treatments, extensive machining, or complex finishing are required even for the prototype, the lead time will be longer. Always confirm lead times with the provider based on your specific requirements.
Conclusion: Launching Lighter, Stronger Satellites with Aluminum AM Brackets
The journey to orbit is demanding, and the economics of space launch place an unrelenting premium on minimizing mass. As we’ve explored, metal additive manufacturing, specifically Laser Powder Bed Fusion of advanced aluminum alloys like AlSi10Mg and the high-performance Scalmalloy®, offers a powerful solution for creating the lightweight, complex, and highly optimized satellite brackets needed for next-generation space missions.
The advantages are compelling: substantial weight reductions achieved through topology optimization and generative design, the ability to consolidate multiple components into single, intricate parts enhancing reliability, and the acceleration of development cycles through rapid prototyping and tool-less production. These benefits translate directly into lower launch costs, increased payload capacity, improved satellite performance, and faster time-to-orbit. While challenges related to process control, post-processing, and quality assurance exist, they are being effectively addressed through advancements in technology, rigorous process validation, and robust quality management systems.
The choice between the workhorse AlSi10Mg, offering a balance of good properties and cost-effectiveness, and the exceptional strength-to-weight ratio and fatigue performance of Scalmalloy® allows engineers to tailor material selection precisely to the specific structural demands and criticality of each bracket application.
However, harnessing the full potential of aluminum AM for mission-critical satellite components requires more than just access to a printer. It demands a holistic approach encompassing expert DfAM guidance, meticulous process control, validated post-processing techniques, stringent quality assurance, and deep materials science knowledge. This underscores the critical importance of selecting the right manufacturing partner.
Companies like Met3dp exemplify the type of partner needed for success in aerospace additive manufacturing. With integrated capabilities spanning advanced powder production using Gas Atomization and PREP technologies, industry-leading printing equipment (SEBM and LPBF), a broad portfolio of advanced aerospace materials, and comprehensive application development services, they offer the expertise and reliability required for demanding projects. Their focus on enabling next-generation manufacturing through cutting-edge systems and powders aligns perfectly with the needs of the evolving space industry. To understand their full capabilities and partnership approach, learn more Über Met3dp.
Die future of satellite manufacturing undoubtedly involves a greater reliance on additive manufacturing. As materials continue to improve, process speeds increase, and design tools become more sophisticated, aluminum AM will play an even larger role in creating lighter, stronger, and more capable satellite structures. By embracing this technology and collaborating with expert metal AM partnership providers, the aerospace industry can continue to push the boundaries of exploration and innovation.
Ready to explore how aluminum additive manufacturing can revolutionize your satellite bracket designs? Contact Met3dp today to discuss your project requirements and discover how their capabilities can power your organization’s additive manufacturing goals.
Teilen auf
MET3DP Technology Co., LTD ist ein führender Anbieter von additiven Fertigungslösungen mit Hauptsitz in Qingdao, China. Unser Unternehmen ist spezialisiert auf 3D-Druckgeräte und Hochleistungsmetallpulver für industrielle Anwendungen.
Fragen Sie an, um den besten Preis und eine maßgeschneiderte Lösung für Ihr Unternehmen zu erhalten!
Verwandte Artikel
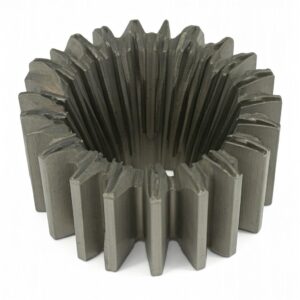
Hochleistungs-Düsenschaufelsegmente: Revolutionierung der Turbineneffizienz mit 3D-Metalldruck
Mehr lesen "Über Met3DP
Aktuelles Update
Unser Produkt
KONTAKT US
Haben Sie Fragen? Senden Sie uns jetzt eine Nachricht! Wir werden Ihre Anfrage mit einem ganzen Team nach Erhalt Ihrer Nachricht bearbeiten.
Holen Sie sich Metal3DP's
Produkt-Broschüre
Erhalten Sie die neuesten Produkte und Preislisten
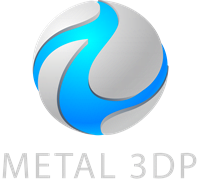
Metallpulver für 3D-Druck und additive Fertigung
UNTERNEHMEN
PRODUKT
cONTACT INFO
- Qingdao Stadt, Shandong, China
- [email protected]
- [email protected]
- +86 19116340731