Precision Instrument Mounts for Spacecraft via 3D Printing
Spis treści
Introduction: The Critical Role of Instrument Mounts in Space Missions
Spacecraft represent the pinnacle of human engineering, venturing into environments far removed from the forgiving conditions on Earth. From Low Earth Orbit (LEO) to the furthest reaches of our solar system, these sophisticated machines carry payloads designed to observe, measure, communicate, and explore. Central to the success of any space mission is the ability of these instruments – the eyes, ears, and senses of the spacecraft – to function flawlessly. This requires not only the instruments themselves to be robust but also relies critically on the structures that hold them: the instrument mounts.
A spacecraft instrument mount is far more than a simple bracket. It is a precision-engineered interface designed to securely attach sensitive equipment, such as cameras, spectrometers, antennas, sensors, or propulsion components, to the main structure of the satellite, probe, lander, or rover. Its fundamental purpose is multifaceted:
- Structural Support: It must bear the static and dynamic loads experienced throughout the mission lifecycle, from ground handling and launch vibrations to in-orbit manoeuvres and potential deployment stresses.
- Precise Alignment: Many instruments, particularly optical systems or directed communication arrays, require exceptionally stable and precise alignment relative to the spacecraft body or specific celestial targets. The mount must maintain this alignment under varying thermal and mechanical conditions.
- Environmental Isolation: It often plays a role in mitigating the harsh space environment’s effects. This can include damping vibrations propagating from the spacecraft structure (e.g., reaction wheels, thruster firings) or providing a degree of thermal isolation or controlled thermal conductivity between the instrument and the spacecraft bus.
The challenges associated with designing and manufacturing these critical space mission hardware components are significant. The space environment is uniquely hostile:
- Ekstremalne temperatury: Components can experience temperature swings of hundreds of degrees Celsius, cycling between direct sunlight and deep shadow. Materials must maintain their structural integrity and dimensional stability across this range, and differential thermal expansion between the mount, instrument, and spacecraft structure must be carefully managed.
- Vibration & Acoustics: The launch phase subjects the entire spacecraft to intense vibrations and acoustic loads. Instrument mounts must be designed to withstand these forces without failing or allowing excessive vibration to damage the sensitive payload.
- Vacuum: The vacuum of space introduces challenges like outgassing, where materials release trapped volatiles that can contaminate sensitive optical surfaces or electronics. Materials must be selected for low outgassing properties. Cold welding can also occur between contacting metal surfaces in a vacuum.
- Radiation: Depending on the orbit or trajectory, components are exposed to various forms of radiation (protons, electrons, cosmic rays) that can degrade material properties over time.
- Mass Constraints: Every gram launched into orbit carries a significant cost penalty. Therefore, achieving the required performance with the minimum possible mass (lightweighting) is a primary driver in inżynieria lotnicza dla satellite hardware.
Traditionally, manufacturing precision mounting structures for spacecraft involved subtractive methods, primarily CNC machining from billet materials like aluminum or titanium alloys. While highly precise, this approach faces limitations, especially as instrument designs become more complex and mass reduction targets become more aggressive. Machining intricate, organically shaped, lightweight structures can be extremely time-consuming, generate significant material waste (buy-to-fly ratio), and may require complex multi-part assemblies with associated fasteners, increasing weight, complexity, and potential failure points. Producing hollow structures or internal features like conformal cooling channels is often impossible.
To jest miejsce wytwarzanie przyrostowe metali (AM), often referred to as metal Drukowanie 3D, emerges as a transformative solution for komponenty statku kosmicznego. Technologies like Laser Powder Bed Fusion (L-PBF) and Electron Beam Melting (EBM) build parts layer by layer directly from digital models using high-performance metal powders. This approach fundamentally changes the design paradigm, enabling the creation of highly optimized, lightweight, and complex instrument mounts that were previously unmanufacturable. Additive manufacturing aerospace applications are rapidly expanding, offering engineers unprecedented freedom to design for function and performance, overcoming many traditional constraints and paving the way for more capable and cost-effective space missions. Companies specializing in these advanced techniques, like Met3dp, provide the crucial expertise and capabilities needed to realize these benefits for demanding inżynieria lotnicza challenges.
Applications and Use Cases: Where are 3D Printed Instrument Mounts Deployed?
The versatility and performance advantages of metal additive manufacturing have led to its adoption across a wide spectrum of spacecraft and mission types for creating sophisticated instrument mounts and payload mounting structures. The ability to tailor designs for specific functional requirements, optimize for weight, and integrate features makes 3D printing particularly attractive for high-value, performance-critical zastosowania lotnicze i kosmiczne. Procurement managers working with defense contractor suppliers and commercial NewSpace ventures are increasingly sourcing these advanced commercial space components.
Here are some key application areas where 3D printed instrument mounts are making a significant impact:
- Optical Payloads:
- Telescopes & Imagers: Maintaining the precise alignment of mirrors, lenses, detectors, and focal plane assemblies is paramount. 3D printed mounts, often utilizing topology optimization, provide exceptional stiffness-to-weight ratios, ensuring stability under thermal loads and micro-vibrations. Materials like Ti-6Al-4V offer low thermal expansion, critical for optical stability. Mounts for large primary or secondary mirrors, complex camera arrays, and support structures for baffles benefit greatly.
- Spectrometers & Interferometers: These instruments rely on precise positioning of gratings, beam splitters, and detectors. AM allows for complex geometries that integrate mounting features, thermal control pathways (like integrated heat pipes or conductive straps), and vibration damping elements directly into the satellite instrument bracket.
- Star Trackers & Sun Sensors: Essential for attitude determination and control, these sensors require stable mounting platforms. AM enables lightweight brackets with optimized geometries that minimize obstruction and maintain alignment accuracy.
- Radio Frequency (RF) Systems:
- Antennas & Feeds: Mounts for various antenna types (parabolic dishes, phased arrays, helical antennas) benefit from AM’s ability to create complex shapes optimized for RF performance and structural integrity. Lightweighting is crucial, especially for large deployable antenna structures. Waveguide components and feed horn supports can be integrated directly into the mounting structure, reducing part count and interfaces.
- Transponders & Amplifiers: These components generate heat and require stable mounting with effective thermal management. 3D printed mounts can incorporate conformal cooling channels or optimized conductive paths to efficiently dissipate heat, improving reliability and performance.
- Sensors & Detectors:
- Environmental Sensors: Instruments measuring magnetic fields, plasma properties, radiation levels, or atmospheric composition often require specific positioning and isolation. 3D printed mounts allow for custom designs tailored to the sensor’s needs, potentially incorporating shielding or specific thermal properties.
- Inertial Measurement Units (IMUs): Gyroscopes and accelerometers within IMUs require extremely stable mounting platforms to ensure accurate navigation and attitude sensing. High-stiffness, low-CTE (Coefficient of Thermal Expansion) 3D printed mounts are advantageous.
- Scientific Probes: Instruments on planetary landers or deep space probes measuring surface properties, seismic activity, or collecting samples need robust and reliable mounts capable of withstanding harsh environments and potential impacts.
- Propulsion & Fluid Systems:
- Thruster Mounts: Small satellite thrusters (cold gas, electric propulsion) require precise alignment and robust mounting. AM can produce brackets with optimized load paths and reduced mass.
- Valve & Tubing Supports: Complex routing of fluid lines can be supported by custom-designed, lightweight 3D printed brackets, consolidating multiple traditional clamps and supports into single components.
Industries and Mission Types:
- Commercial Space (NewSpace): Large satellite constellations for communications (Starlink, OneWeb) or Earth observation rely heavily on reducing launch costs through lightweighting. AM enables rapid production of optimized components like instrument mounts for hundreds or thousands of satellites. CubeSats and SmallSats also benefit significantly from the miniaturization and integration possibilities offered by 3D printing.
- Government & Defense: Agencies like NASA, ESA, JAXA, and defense organizations utilize AM for unique, high-performance hardware for flagship science missions (e.g., space telescopes, Mars rovers, deep space probes) and reconnaissance or communication satellites. The ability to create bespoke designs and potentially reduce lead times for critical missions is highly valuable. As a key defense contractor supplier consideration, secure and reliable manufacturing processes are paramount.
- Research Institutions: Universities and research labs developing experimental payloads or CubeSat missions leverage AM for its accessibility, speed in prototyping, and ability to create custom hardware for specific scientific objectives.
Specific examples might include topology-optimized brackets for holding scientific instruments on the Mars Perseverance rover, lightweight antenna supports for communication satellites, or complex optical benches for Earth observation imagers where stability is paramount. The trend is clear: as mission requirements become more demanding and launch costs remain a critical factor, metal additive manufacturing aerospace solutions like 3D printed instrument mounts will play an increasingly vital role across all sectors of the space industry.
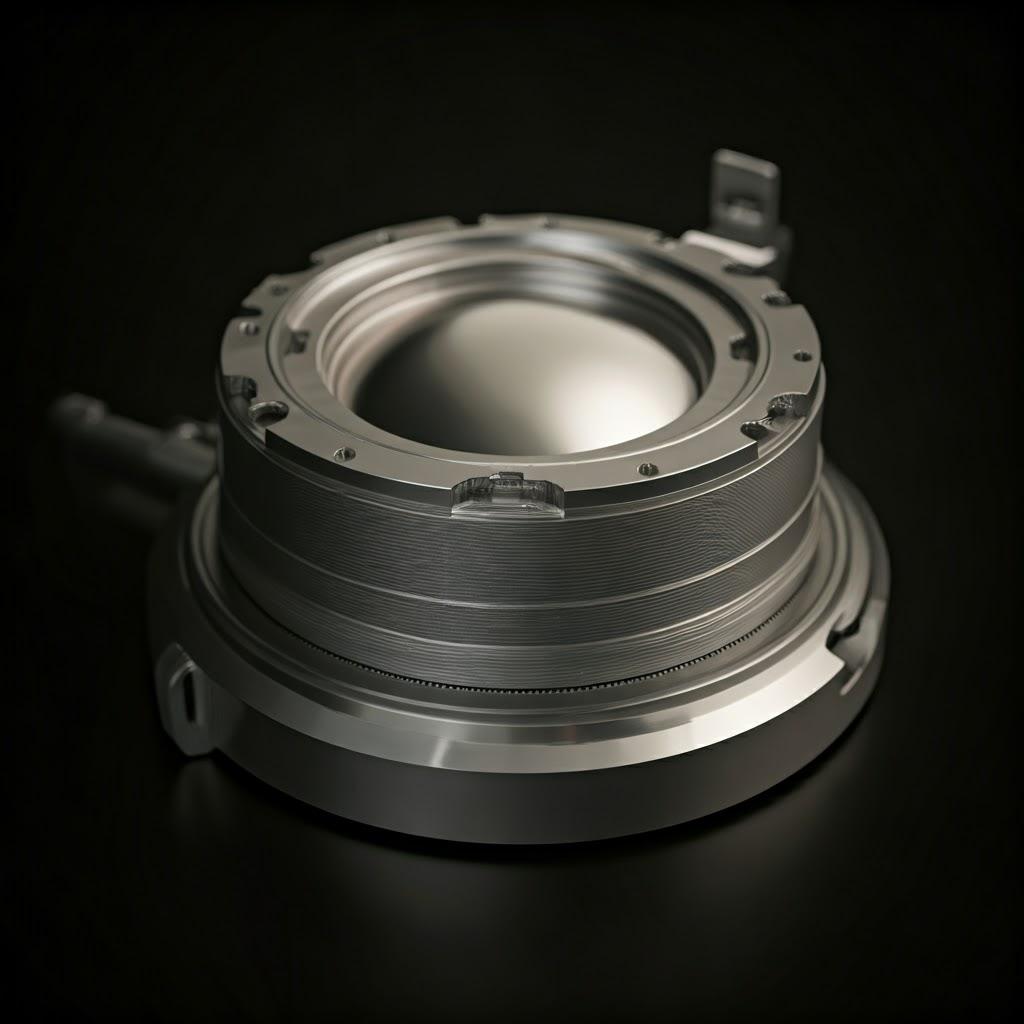
Why Metal 3D Printing for Spacecraft Instrument Mounts? Unlocking Performance Advantages
Przyjęcie metal 3D printing aerospace technologies for manufacturing spacecraft instrument mounts is driven by a compelling set of advantages over traditional subtractive methods like CNC machining. These benefits translate directly into improved performance, reduced costs, faster development cycles, and enhanced mission capabilities. For engineers striving to push the boundaries of inżynieria lotnicza and procurement managers seeking cost-effective, high-value komponenty statku kosmicznego, understanding these advantages is crucial.
Here’s a breakdown of why additive manufacturing is revolutionizing the production of these critical parts:
- Unprecedented Lightweighting:
- Topology Optimization: AM enables the practical application of topology optimization algorithms. These tools determine the most efficient material distribution within a defined design space to meet specific load and stiffness requirements. The result is often organic, skeletal structures that remove significant amounts of non-essential material, drastically reducing part weight compared to traditionally machined components designed with simpler geometries. This directly lowers launch costs (estimated at thousands to tens of thousands of dollars per kilogram) and can allow for more payload capacity.
- Struktury kratowe: AM allows for the incorporation of complex internal lattice structures. These periodic cellular materials offer exceptional stiffness-to-weight ratios, energy absorption capabilities (for vibration damping), and can facilitate heat transfer. They are virtually impossible to create using subtractive methods.
- Geometric Complexity & Design Freedom:
- “Complexity is Free”: Unlike machining, where complex features increase machining time and cost significantly, the layer-by-layer nature of AM means intricate designs (within process limits) do not inherently add substantial cost or time penalties. This liberates engineers to design parts optimized for function, rather than being constrained by manufacturability via traditional means.
- Integrated Features: Functional features like cable routing channels, fluid pathways (conformal cooling/heating), integrated fasteners, kinematic mounting points, and complex mating interfaces can be built directly into the mount structure.
- Konsolidacja części:
- Reduced Assembly: Multiple individual components that would traditionally be machined separately and then assembled using fasteners can often be redesigned and printed as a single, monolithic part.
- Korzyści: This significantly reduces assembly time and labor costs, eliminates potential failure points associated with joints and fasteners (improving reliability), and further contributes to weight reduction by removing the mass of the fasteners themselves. This is a key driver for part consolidation AM strategies.
- Enhanced Performance Characteristics:
- Vibration Damping: The ability to incorporate optimized geometries and lattice structures allows for mounts designed with specific vibration damping characteristics, better protecting sensitive instruments from launch loads and spacecraft-induced vibrations.
- Zarządzanie temperaturą: AM enables the creation of mounts with integrated, highly efficient thermal control features. Conformal cooling channels following the contours of heat-generating components or optimized conductive pathways can be designed directly into the part, improving thermal stability and instrument performance. Materials like Scalmalloy® offer good thermal conductivity for heat dissipation.
- Tailored Stiffness: Topology optimization allows engineers to precisely place material where it’s needed most, achieving exceptional stiffness in critical directions while minimizing mass elsewhere.
- Rapid Prototyping, Iteration, and Reduced Lead Times:
- Prędkość: AM allows for the direct production of parts from CAD models, bypassing the need for traditional tooling (jigs, fixtures, molds). This drastically shortens the lead time from design finalization to having a physical part in hand, often reducing it from weeks or months to days.
- Faster Iteration: Design modifications can be implemented quickly in the CAD model and a new part printed rapidly, enabling faster design-build-test cycles during development. This accelerates innovation and allows for more optimized final designs. This advantage in rapid prototyping aerospace is invaluable.
- Produkcja na żądanie: AM offers the potential for on-demand production, reducing the need for large inventories and mitigating supply chain risks associated with traditional manufacturing dependencies.
- Advanced Material Utilization:
- Optimized Materials: AM processes are compatible with advanced, high-performance alloys specifically suited for the space environment, such as high-strength aluminum alloys (Scalmalloy®) and titanium alloys (Ti-6Al-4V). These space-grade materials offer superior specific strength, corrosion resistance, and stability across temperature extremes.
- Zmniejszona ilość odpadów: While powder management is critical, AM processes generally produce less material waste compared to subtractive machining, especially for complex parts where the buy-to-fly ratio (weight of raw material purchased vs. weight of the final part) can be very high for machining.
Comparison: AM vs. Traditional Machining for Instrument Mounts
Cecha | Wytwarzanie przyrostowe metali (AM) | Tradycyjna obróbka CNC |
---|---|---|
Lekkość | Excellent (Topology Optimization, Lattices) | Limited (Constrained by billet form, access) |
Złożoność | High geometric freedom, “Complexity is Free” | Cost/Time increases significantly |
Konsolidacja części | Excellent capability | Limited, requires assembly |
Integrated Features | High (Cooling channels, routing, etc.) | Difficult or impossible |
Czas realizacji | Fast (Days/Weeks) | Slower (Weeks/Months, tooling dependent) |
Odpady materiałowe | Lower (Powder bed reuse, near-net shape) | Higher (Significant swarf/chip generation) |
Koszt oprzyrządowania | None (Direct digital manufacturing) | Can be significant (jigs, fixtures) |
Design Iteration | Rapid | Wolniej |
Achievable Precision | Good (Often requires post-machining for critical features) | Doskonały |
Wykończenie powierzchni | Fair to Good (As-built), requires finishing | Doskonały |
Export to Sheets
While traditional machining still excels in achieving the very highest levels of surface finish and tolerance directly, metal AM provides a powerful alternative, particularly when design complexity, weight reduction, and integrated functionality are primary drivers. Often, a hybrid approach is used, where the bulk of the complex structure is 3D printed, followed by targeted CNC machining of critical interfaces to achieve final tolerances. Partnering with an experienced AM provider like Met3dp ensures that the optimal manufacturing strategy is employed to leverage these compelling advantages for your specific aerospace application.
Recommended Materials: Scalmalloy® and Ti-6Al-4V for Extreme Environments
Selecting the right material is fundamental to the success of any spacecraft component, especially for precision instrument mounts operating under extreme conditions. The material must provide the necessary structural integrity, stability, and environmental resistance while adhering to stringent mass limitations. Metal additive manufacturing opens the door to utilizing advanced alloys that are particularly well-suited for these demanding zastosowania lotnicze i kosmiczne. Two standout materials processed via Powder Bed Fusion (PBF) techniques like L-PBF and EBM are Scalmalloy® (an aluminum alloy) and Ti-6Al-4V (a titanium alloy). Understanding their unique properties and benefits is crucial for engineers and procurement managers specifying space-grade materials.
Scalmalloy® (Al-Mg-Sc Alloy): The High-Performance Aluminum
Scalmalloy® is a patented high-strength aluminum-magnesium-scandium alloy specifically designed for additive manufacturing. Developed by APWorks (an Airbus subsidiary), it has rapidly gained traction in aerospace due to its exceptional combination of properties, often exceeding those of traditional high-strength aerospace aluminum alloys.
- Kluczowe właściwości:
- High Specific Strength: Offers a strength-to-weight ratio significantly higher than traditional aerospace aluminum alloys (e.g., AlSi10Mg) and comparable to some titanium grades, making it ideal for lightweighting.
- Excellent Ductility & Toughness: Unlike many high-strength aluminum alloys, Scalmalloy® retains good ductility and fracture toughness, even at cryogenic temperatures, enhancing structural reliability.
- Dobra spawalność: Can be effectively welded, which is advantageous for potential post-processing or assembly operations, although AM often aims to eliminate such needs through consolidation.
- Odporność na korozję: Exhibits good resistance to corrosion.
- Processability via L-PBF: Well-characterized and optimized for Laser Powder Bed Fusion processes.
- Mikrostruktura: Exhibits a fine-grained microstructure after printing and heat treatment, contributing to its superior mechanical properties.
- Benefits for Instrument Mounts:
- Significant Weight Savings: Its primary advantage. Enables the design of mounts with thin walls and complex, topology-optimized geometries that drastically reduce mass compared to parts made from conventional aluminum or even titanium in some stiffness-driven designs.
- Stiffness-Critical Applications: Where maintaining shape and alignment under load is crucial (e.g., optical benches, mirror mounts), its high specific stiffness is highly beneficial.
- Cryogenic Performance: Suitable for missions involving exposure to very low temperatures.
- Uwagi dotyczące przetwarzania: Requires careful control of L-PBF parameters and specific post-processing heat treatments (solution annealing and aging) to achieve optimal properties. Susceptible to hot cracking if process parameters are not optimized.
Ti-6Al-4V (Grade 5 Titanium): The Aerospace Workhorse
Ti-6Al-4V (Titanium-6% Aluminum-4% Vanadium), often referred to as Grade 5 titanium, is arguably the most widely used titanium alloy in aerospace and medical applications, and it translates well to additive manufacturing via both L-PBF and EBM (Electron Beam Melting).
- Kluczowe właściwości:
- Wysoki stosunek wytrzymałości do wagi: Excellent specific strength, maintained up to moderately elevated temperatures (around 300-400°C).
- Wyjątkowa odporność na korozję: Highly resistant to corrosion in various environments, including oxidizing acids and chloride solutions, crucial for long-duration missions and preventing contamination.
- Niska rozszerzalność cieplna: Has a relatively low Coefficient of Thermal Expansion (CTE), which is highly advantageous for maintaining dimensional stability in optical systems or precision alignments during thermal cycling.
- Doskonała biokompatybilność: While less relevant for instrument mounts than for medical implants, it indicates the material’s inert nature.
- Good Fatigue Life & Fracture Toughness: Robust performance under cyclic loading conditions.
- Processability via L-PBF & EBM: Can be processed effectively using both major PBF technologies. EBM often results in lower residual stress but typically rougher surface finish compared to L-PBF.
- Benefits for Instrument Mounts:
- Strength-Driven Designs: Ideal when high absolute strength, fatigue resistance, and fracture toughness are the primary requirements.
- Stabilność termiczna: Its low CTE makes it the preferred choice for mounts requiring extreme dimensional stability across temperature ranges, such as those used in high-precision optical assemblies.
- Harsh Environments: Superior corrosion resistance makes it suitable for applications potentially exposed to residual propellants or specific planetary atmospheres.
- Elevated Temperature Applications: Maintains strength better than aluminum alloys at higher operating temperatures.
- Uwagi dotyczące przetwarzania: Titanium powders are reactive and require careful handling in inert atmospheres to prevent oxygen pickup, which can degrade mechanical properties. Post-processing often includes stress relief and Hot Isostatic Pressing (HIP) to reduce internal porosity and improve fatigue properties, especially for flight-critical parts.
Material Selection: A Comparative Glance
Nieruchomość | Scalmalloy® | Ti-6Al-4V (klasa 5) | Relevance for Instrument Mounts |
---|---|---|---|
Gęstość | ~2.67 g/cm³ | ~4,43 g/cm³ | Lower density favors Scalmalloy® for pure lightweighting. |
Yield Strength (Typical AM) | ~450-500 MPa | ~900-1100 MPa (Heat Treated/HIPed) | Ti-6Al-4V offers higher absolute strength. |
Specyficzna siła | Bardzo wysoka | Bardzo wysoka | Both excellent; Scalmalloy® slightly better in some conditions. |
Stiffness (Young’s Modulus) | ~70 GPa | ~110-115 GPa | Ti-6Al-4V is significantly stiffer. |
Specific Stiffness | Bardzo wysoka | Wysoki | Scalmalloy® excels where stiffness-per-unit-mass is critical. |
Max Service Temp. | ~125-150 °C | ~350-400 °C | Ti-6Al-4V suitable for higher temperature operation. |
Thermal Expansion (CTE) | ~21-23 µm/m·K | ~8.6-9.2 µm/m·K | Ti-6Al-4V provides superior dimensional stability with temp changes. |
Przewodność cieplna | ~110-120 W/m·K | ~6.7-7.5 W/m·K | Scalmalloy® is much better for heat dissipation if needed. |
Koszt | High (Scandium content) | High (Titanium base, processing) | Both are premium materials; costs depend on application/volume. |
Export to Sheets
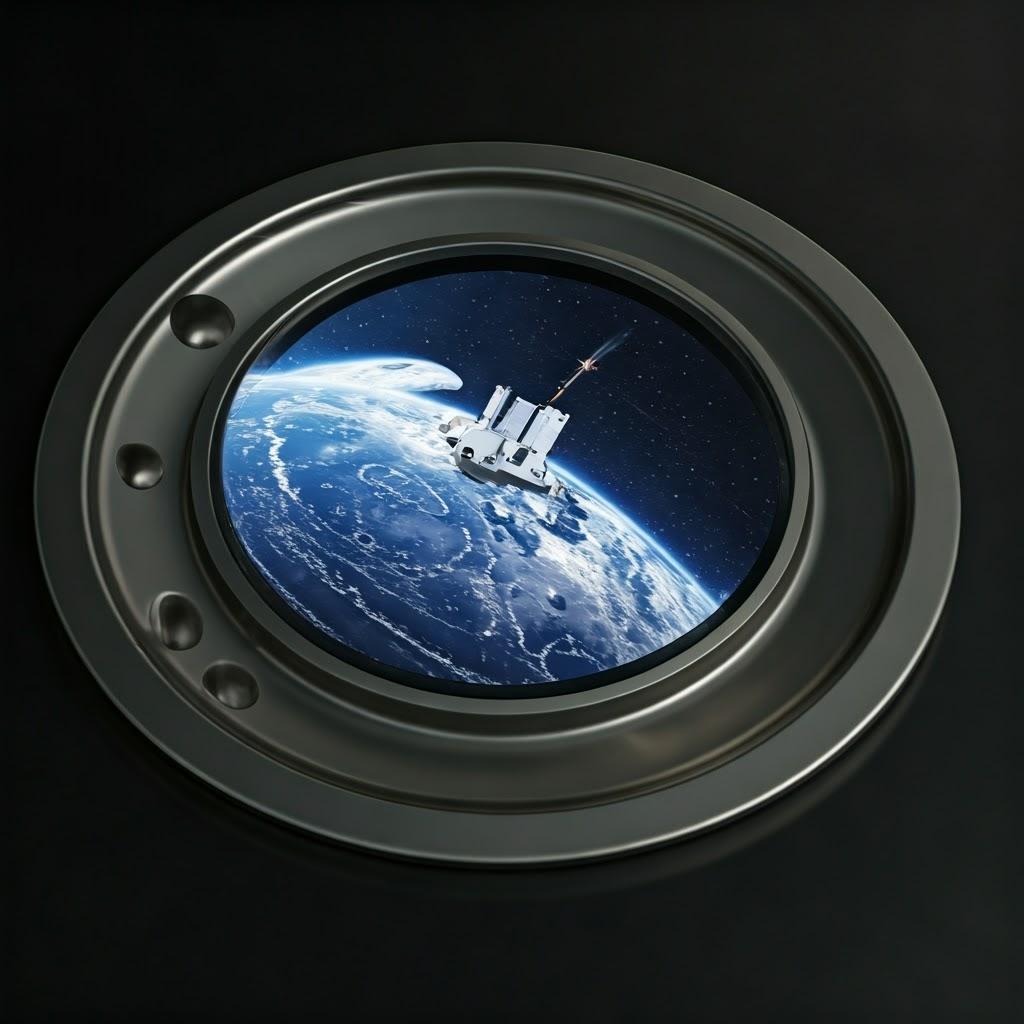
The Importance of Powder Quality: The Met3dp Advantage
The ultimate performance of a 3D printed component, whether Scalmalloy®, Ti-6Al-4V, or other advanced alloys like Inconel or specialized steels, fundamentally depends on the quality of the input metal powder. Factors like particle size distribution (PSD), sphericity, flowability, purity, and internal porosity directly impact the density, mechanical properties, and surface finish of the final part.
This is where partnering with a vertically integrated provider like Met3dp offers significant advantages. Met3dp not only possesses expertise in druk 3D z metalu processes like SEBM (Selective Electron Beam Melting – similar to EBM) but also specializes in producing the feedstock itself. Our company employs industry-leading powder production technologies:
- Gas Atomization (GA): Utilizes high-pressure inert gas jets to break up a stream of molten metal into fine, spherical droplets that solidify rapidly. Our advanced gas atomization equipment uses unique nozzle and gas flow designs to produce metallic spheres with exceptional sphericity and flowability, critical for uniform layer spreading in PBF processes.
- Proces plazmowej elektrody rotacyjnej (PREP): This method uses a rapidly rotating electrode bar made of the desired alloy, which is melted at the tip by a plasma torch. Centrifugal force ejects molten droplets that solidify in flight into highly spherical powders with very low internal porosity and satellite content, ideal for the most demanding applications.
By controlling the powder production process, Met3dp ensures a consistent supply of Wysokiej jakości proszki metali optimized for additive manufacturing, including alloys like TiNi, TiTa, TiAl, TiNbZr, CoCrMo, various stainless steels, and superalloys, alongside standards like Ti-6Al-4V. This commitment to powder quality, combined with decades of collective expertise in metal AM, allows us to deliver komponenty lotnicze with superior density, mechanical properties, and reliability, enabling our partners to fully leverage the benefits of materials like Scalmalloy® and Ti-6Al-4V for their critical spacecraft instrument mounts. Choosing the right aerospace metal powders supplier is as critical as choosing the printing service itself.
Design for Additive Manufacturing (DfAM): Optimizing Instrument Mounts for 3D Printing
Transitioning from traditional design constraints to leveraging the full potential of metal additive manufacturing requires a shift in thinking. Design for Additive Manufacturing (DfAM) is not simply about making existing designs printable; it’s about fundamentally rethinking component geometry to maximize the benefits of the layer-by-layer fabrication process, particularly crucial for high-stakes zastosowania lotnicze i kosmiczne like spacecraft instrument mounts. Applying DfAM aerospace principles enables engineers to achieve superior performance, reduce weight, consolidate parts, and shorten development cycles. Ignoring DfAM often leads to suboptimal results, increased post-processing challenges, higher costs, and potentially failed prints.
Here are key DfAM principles and considerations for optimizing instrument mounts for metal 3D printing aerospace processes like L-PBF and EBM:
- Embrace Topology Optimization and Generative Design:
- Concept: These computational tools are star players in DfAM. Engineers define the design space (maximum allowable volume), load cases (forces, pressures, thermal loads), constraints (keep-out zones, mounting points), and performance objectives (minimize mass, maximize stiffness). The topology optimization software then iteratively removes material from non-critical areas, revealing the most efficient load-bearing structure. Generative design often explores multiple optimized solutions based on parameters.
- Outcome: Results are often organic, “bionic” looking structures that are highly efficient in their material usage, leading to significant weight savings (often 20-50% or more) while meeting or exceeding performance requirements. This is ideal for reducing launch costs associated with satellite hardware.
- Consideration: Optimized designs can be complex and may require careful analysis to ensure manufacturability (e.g., avoiding features too thin to print reliably).
- Leverage Lattice Structures Strategically:
- Concept: AM allows the incorporation of intricate internal lattice or cellular structures. These can be uniform or graded (varying density) and come in various cell types (cubic, octet-truss, gyroid, etc.), each offering different mechanical, thermal, or flow properties.
- Applications for Mounts:
- Lightweight Infilling: Replacing solid volumes with low-density lattices maintains structural integrity while drastically reducing mass.
- Vibration Damping: Certain lattice topologies excel at absorbing vibrational energy, helping to isolate sensitive instruments.
- Zarządzanie temperaturą: Lattices can be designed to enhance or impede heat transfer, or facilitate fluid flow for active cooling.
- Consideration: Requires specialized design software and careful consideration of printability (minimum strut size, powder removal from internal cavities). Lattice structure design is an advanced DfAM technique.
- Design for Minimum Support Structures:
- Wyzwanie: Support structures are often necessary in PBF processes to anchor the part to the build plate, support overhanging features (typically angles below 45 degrees from horizontal), and aid heat dissipation to prevent warping. However, supports consume extra material, add print time, and require significant post-processing effort for removal, which can damage surfaces and be difficult in complex internal areas.
- Strategies:
- Self-Supporting Angles: Design overhangs to be above the critical angle (often ~45°, but material/machine dependent) whenever possible.
- Feature Orientation: Cleverly orienting the part on the build platform can transform overhangs into self-supporting features. This is a critical step in the build orientation strategy.
- Incorporate Sacrificial Features: Sometimes, designing features like chamfers or fillets instead of sharp horizontal overhangs can make them self-supporting.
- Internal Channels: Design channels with diamond or teardrop cross-sections instead of circular ones to make the “roof” self-supporting.
- Goal: Minimize reliance on supports, especially complex internal ones, to reduce cost, lead time, and post-processing complexity.
- Optimize Part Orientation:
- Kompromisy: The orientation of the instrument mount within the build chamber significantly impacts several factors:
- Wykończenie powierzchni: Downward-facing surfaces and surfaces directly supported often have poorer finish (higher Ra) than upward-facing or vertical walls. Critical surfaces should ideally be oriented for best finish.
- Właściwości mechaniczne: Anisotropy (direction-dependent properties) can exist in AM parts, though often minimized with proper parameters and post-processing (like HIP). Orientation can influence strength and ductility relative to primary load directions.
- Support Requirements: As mentioned, orientation is key to minimizing supports.
- Build Time & Cost: Taller orientations generally increase build time. Fitting more parts onto a single build plate reduces per-part cost.
- Distortion: Orientation affects thermal gradients and potential for warping.
- Best Practice: Use simulation tools and process expertise (like that offered by Met3dp) to determine the optimal orientation balancing these competing factors based on the specific part requirements.
- Kompromisy: The orientation of the instrument mount within the build chamber significantly impacts several factors:
- Adhere to Process-Specific Design Rules:
- Minimum Wall Thickness: Parts need sufficient thickness to be handled and function; features too thin may warp or not resolve properly during printing. Typical minimums are often in the 0.4mm – 1.0mm range, depending on the machine, material, and feature height.
- Hole Sizes & Diameters: Small holes (e.g., < 0.5mm) may seal shut during printing or be difficult to clear of powder. Hole verticality affects roundness.
- Aspect Ratios: Tall, thin walls are prone to distortion or failure during printing.
- Trapped Volumes & Powder Removal: Avoid designing enclosed hollow sections from which unmelted powder cannot be removed post-print. Design escape holes strategically.
- Understanding Machine Limits: Be aware of the specific build volume, laser/beam spot size, and layer thickness capabilities of the AM system being used.
- Integrate Functionality Directly:
- Part Consolidation Mindset: Actively look for opportunities to combine multiple traditionally separate parts (brackets, clamps, thermal straps, housings) into a single, multifunctional printed component.
- Przykłady: Design integrated channels for wiring harnesses, conformal cooling/heating channels that precisely follow instrument contours, built-in kinematic mounting features for precise alignment, or specific surface textures for thermal radiation properties.
- Design for Post-Processing:
- Machining Allowances: If tight tolerances or specific surface finishes are required on certain features (e.g., mating interfaces, bearing seats), add extra “sacrificial” material (machining stock, typically 0.5-2mm) to those areas in the design phase. This ensures enough material is present for clean-up via CNC machining after printing and heat treatment.
- Support Removal Access: Ensure adequate physical and visual access for removing support structures, especially internal ones. Avoid designs where supports are effectively trapped.
- Inspection Features: Consider adding datum features or reference points to facilitate CMM inspection later.
By diligently applying these additive manufacturing design rules, engineers can unlock the true potential of metal AM for creating next-generation spacecraft instrument mounts that are lighter, stronger, more functional, and often faster to produce than their traditionally manufactured counterparts. Collaborating with experienced AM service providers like Met3dp early in the design phase can provide invaluable feedback and ensure designs are optimized for successful production.
Achieving Precision: Tolerance, Surface Finish, and Dimensional Accuracy in Metal AM
While metal additive manufacturing offers unparalleled design freedom, a common question from engineers and procurement managers, especially those accustomed to the precision of CNC machining, concerns the achievable levels of tolerance, surface finish, and overall dimensional accuracy. Understanding the capabilities and limitations of metal 3D printing tolerances and finish is crucial for setting realistic expectations and ensuring components meet the stringent requirements of aerospace component inspection and function.
Dimensional Accuracy & Tolerances:
- General Capabilities: Metal Powder Bed Fusion (PBF) processes like L-PBF and EBM can achieve reasonably good dimensional accuracy directly from the machine. Typical tolerances are often cited in the range of ±0.1 mm to ±0.3 mm for smaller features (e.g., up to 50-100 mm), with potentially larger deviations (e.g., ±0.1% to ±0.2% of length) for larger dimensions. Some providers may quote tolerances relative to standards like ISO 2768 (medium ‘m’ or coarse ‘c’ classes) for general dimensions, but specific achievable tolerances depend heavily on the factors below.
- Factors Influencing Accuracy:
- Thermal Effects: The repeated heating and cooling during the layer-wise process induce thermal stresses. As the part cools, these stresses can cause shrinkage and warping, leading to dimensional deviations. This is a primary challenge affecting dimensional accuracy additive manufacturing.
- Part Geometry & Size: Larger parts and complex geometries with varying cross-sections are generally more prone to distortion. Tall, thin features are also challenging.
- Materiał: Different materials have different thermal properties (conductivity, expansion) affecting stress buildup and shrinkage.
- Support Strategy: The type, density, and location of support structures significantly impact heat dissipation and part stability during the build, influencing final accuracy. Improper supports can exacerbate warping.
- Machine Calibration & Condition: Laser/beam alignment, scanner accuracy, powder layer consistency, and overall machine health are critical. Providers like Met3dp emphasize rigorous calibration and maintenance for their industry-leading accuracy printers.
- Orientacja budynku: As discussed in DfAM, orientation affects thermal history and support needs, thereby impacting accuracy.
- Przetwarzanie końcowe: Stress relief heat treatments can cause minor dimensional changes. Support removal can also affect nearby surfaces.
- Achieving Tighter Tolerances: For critical interfaces, mounting holes, or features requiring tolerances tighter than the standard AM process capability (e.g., tighter than ±0.1 mm), post-process CNC machining is typically required. This hybrid approach leverages AM’s ability to create the complex overall shape efficiently and machining’s precision for final finishing of critical features. Designing with appropriate machining allowances (stock) is essential for this workflow.
Surface Finish (Roughness):
- As-Built Surface Finish: The surface finish, typically quantified by the average roughness (Ra), of as-built metal AM parts is generally rougher than machined surfaces.
- L-PBF: Typically achieves Ra values ranging from 6 µm to 15 µm (or higher) depending on material, orientation, parameters, and surface type (up-skin, down-skin, vertical).
- EBM: Generally produces rougher surfaces than L-PBF, often with Ra values from 20 µm to 40 µm or more, due to larger powder particles and higher beam energy causing more melt pool spatter and partially sintered particles adhering to surfaces.
- Factors Influencing Surface Finish:
- Grubość warstwy: Thinner layers generally result in smoother surfaces, especially on sloped features, but increase build time.
- Powder Particle Size: Finer powders typically lead to smoother finishes. Met3dp’s focus on powder quality using advanced atomization contributes to better achievable finishes.
- Laser/Beam Parameters: Energy input, scan speed, and strategy affect melt pool dynamics and surface texture.
- Orientation: Upward-facing surfaces and vertical walls tend to be smoother than downward-facing surfaces (supported) or heavily sloped surfaces due to the “stair-stepping” effect inherent in layer-based processes.
- Poprawa wykończenia powierzchni: If a smoother surface finish metal AM part is required than what’s achievable as-built, various post-processing techniques are employed:
- Abrasive Blasting (Bead/Sand Blasting): Provides a uniform matte finish, effective for removing partially sintered particles but generally increases Ra slightly compared to the best as-built vertical walls.
- Tumbling/Vibratory Finishing: Uses abrasive media in a rotating or vibrating drum to smooth surfaces and edges, suitable for batches of smaller parts.
- CNC Machining: Offers the best control for achieving smooth, precise surfaces on specific features.
- Polishing (Manual or Automated): Can achieve very low Ra values (mirror finish) but is often labor-intensive and typically reserved for specific functional requirements (e.g., optical surfaces, sealing faces).
- Electropolishing: An electrochemical process that preferentially removes peaks, smoothing the surface while also improving cleanability and corrosion resistance (common for Ti-6Al-4V).
- Abrasive Flow Machining (AFM): Flows abrasive putty through internal channels or over external surfaces to achieve fine finishes in hard-to-reach areas.
Metrology and Inspection:
Given the process variability and the criticality of spacecraft components, rigorous inspection is non-negotiable.
- Coordinate Measuring Machines (CMM): Touch probes provide highly accurate dimensional measurements of specific features and datum points. Essential for verifying critical tolerances after printing and post-machining.
- 3D Scanning (Laser or Structured Light): Captures the full 3D geometry of the part, allowing comparison to the original CAD model (geometric dimensioning and tolerancing – GD&T analysis) and identifying overall distortion or deviation across complex surfaces. Faster than CMM for overall form but generally less accurate for individual feature measurements.
- Computed Tomography (CT) Scanning: An invaluable non-destructive testing (NDT) method for AM parts. X-rays are used to create a 3D reconstruction of the part’s internal structure, allowing for:
- Internal Geometry Verification: Checking complex internal channels or features.
- Porosity Detection: Identifying and quantifying internal voids or lack-of-fusion defects that could compromise structural integrity.
- Dimensional Analysis: Measuring internal and external features without sectioning the part.
- Pomiar chropowatości powierzchni: Stylus profilometers or optical profilometers are used to quantify the Ra or other surface texture parameters on specified surfaces.
In summary, while metal AM may not match the inherent micron-level precision of machining across all features directly from the machine, it offers good baseline accuracy and finish. Understanding the process capabilities, applying DfAM principles, and planning for appropriate post-processing (machining, finishing) and rigorous inspection allows manufacturers to reliably achieve the demanding precision required for flight-critical spacecraft instrument mounts. Partnering with knowledgeable providers who maintain tight process control and offer comprehensive metrology is key for aerospace component inspection success.
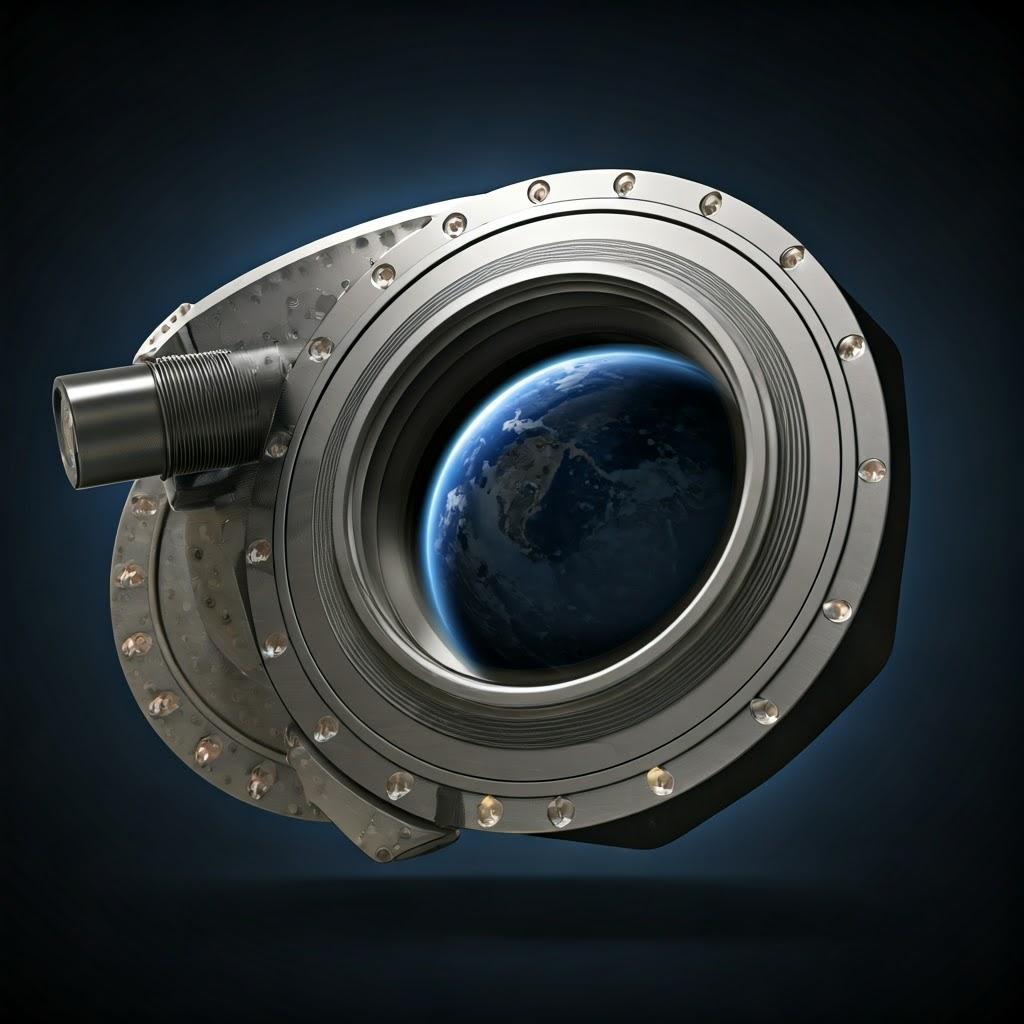
Post-Processing Pathways: Refining 3D Printed Instrument Mounts for Flight
Producing a metal part layer by layer via additive manufacturing is often just the first major step. For demanding applications like spacecraft instrument mounts, especially those deemed flight-critical, a series of post-processing metal AM steps are typically required to transform the as-built component into a finished, flight-ready article. These steps are crucial for relieving internal stresses, improving material properties, achieving final dimensional tolerances and surface finish, removing temporary structures, and ensuring cleanliness. Understanding these pathways is essential for planning production timelines and costs.
Here are the common post-processing steps for 3D printed Scalmalloy® and Ti-6Al-4V instrument mounts:
- Stress Relief Heat Treatment:
- Why: The rapid heating and cooling cycles inherent in PBF processes create significant residual stresses within the printed part. These stresses can cause distortion during or after the build (especially upon removal from the build plate) and can negatively impact mechanical performance (e.g., reducing fatigue life).
- How: The part, often while still attached to the build plate, is heated in a controlled atmosphere furnace (inert gas like Argon for Titanium, sometimes air or vacuum for Aluminum depending on the exact cycle) to a specific temperature below the alloy’s transformation or aging temperatures. It’s held at this temperature for a set duration (typically 1-4 hours) and then slowly cooled. This allows internal stresses to relax without significantly altering the microstructure.
- Outcome: Improved dimensional stability, reduced risk of cracking, preparation for subsequent steps like HIP or machining. This is almost always the first thermal step in heat treatment additive manufacturing.
- Removal from Build Plate & Support Structure Removal:
- Why: The part is built attached to a thick metal plate and uses support structures for anchoring and overhangs. These must be removed.
- How:
- Build Plate Removal: Typically done using wire Electrical Discharge Machining (EDM) or a bandsaw to cut the part free from the base plate.
- Usuwanie wsparcia: This can be one of the most labor-intensive steps, especially for complex parts or strong materials like Titanium. Methods include:
- Manual Breaking/Cutting: For easily accessible, lightweight supports.
- Machining (Milling/Grinding): For bulk removal or precise removal near critical surfaces.
- Wire EDM: Precise cutting for difficult-to-reach supports.
- Specialized Tools: Pliers, chisels, rotary tools.
- Wyzwania: Support removal can potentially damage the part surface if not done carefully. Accessing internal supports can be very difficult, highlighting the importance of DfAM to minimize them. Support removal AM cost and time should not be underestimated.
- Prasowanie izostatyczne na gorąco (HIP):
- Why: While process optimization aims to produce fully dense parts (>99.5% density), microscopic internal pores (due to trapped gas or incomplete fusion) can sometimes remain. These pores act as stress concentrators and can significantly degrade fatigue life and fracture toughness, which is unacceptable for critical components.
- How: The HIP process metal parts involves subjecting the components to both high temperature (below melting point) and high isostatic pressure (typically 100-200 MPa) using a high-purity inert gas (usually Argon) within a specialized pressure vessel. The combination of heat and pressure causes the material to plastically deform and creep on a microscopic level, effectively closing and diffusion bonding internal voids.
- Outcome: Significantly improved material density (approaching 100%), enhanced fatigue strength, increased ductility and toughness, reduced property scatter, leading to more reliable and predictable performance. Often mandatory for flight-critical Ti-6Al-4V parts. May also be required for Scalmalloy® in demanding applications.
- Further Heat Treatments (Solutionizing & Aging):
- Why: To achieve the final desired microstructure and peak mechanical properties (strength, hardness) for specific alloys like Scalmalloy® and Ti-6Al-4V. The as-built or HIPed microstructure might not be optimal.
- How:
- Wyżarzanie roztworu: Heating the alloy to a high temperature to dissolve precipitates and create a homogeneous solid solution, followed by rapid quenching.
- Aging (Precipitation Hardening): Reheating the solutionized part to an intermediate temperature for a specific time, allowing controlled precipitation of fine secondary phases within the metal matrix. These precipitates impede dislocation movement, significantly increasing strength and hardness.
- Outcome: Tailored mechanical properties meeting the design specifications. Specific temperatures and times are critical and alloy-dependent.
- CNC Machining:
- Why: To achieve tight tolerances (typically better than ±0.1 mm), precise geometric features (e.g., flatness, perpendicularity), and smooth surface finishes on critical interfaces that cannot be achieved by the AM process alone.
- How: Using standard CNC milling, turning, or grinding machines to remove the pre-designed machining stock from specific surfaces (e.g., mounting pads, bolt holes, mating faces, bearing bores). Careful fixture design is needed to hold the potentially complex AM part securely without distortion.
- Outcome: Final dimensional accuracy and surface finish on critical features, ensuring proper assembly and function. CNC machining 3D prints is a common hybrid manufacturing approach.
- Wykończenie powierzchni:
- Why: To achieve specific surface roughness (Ra), aesthetic appearance, or prepare the surface for coatings.
- How: As described previously: bead blasting, tumbling, polishing, electropolishing, AFM, depending on the requirements and part geometry. Selection depends on whether a uniform matte finish is needed, if specific Ra values must be met, or if very smooth surfaces are required for sealing or optical reasons.
- Outcome: Desired surface texture and cleanliness. Aerospace surface finishing often requires specific procedures and controls.
- Cleaning & Passivation:
- Why: Ensuring space-grade cleanliness is paramount to prevent outgassing or contamination of sensitive instruments or spacecraft systems. Residual oils, metallic debris, or embedded contaminants must be removed. Passivation (especially for Titanium) enhances the natural protective oxide layer.
- How: Multi-stage cleaning processes involving detergents, solvents, ultrasonic baths, and rinses with high-purity water or specific fluids (e.g., isopropyl alcohol). Passivation typically involves controlled exposure to specific acid solutions (like nitric acid for Ti). Procedures must adhere to strict aerospace standards (e.g., NASA specifications).
- Outcome: A clean, passivated, flight-ready surface meeting mission requirements for space component cleaning.
The specific sequence and necessity of these post-processing steps depend heavily on the material, application requirements (criticality, tolerances, loads), and the chosen AM process. Thorough planning and coordination between design engineers, the AM service provider (like Met3dp with its comprehensive solutions approach), and post-processing specialists are essential for successfully navigating these pathways and delivering reliable, high-performance spacecraft instrument mounts.
Overcoming Challenges in Additive Manufacturing for Spacecraft Components
While metal additive manufacturing presents enormous opportunities for aerospace, particularly for complex components like instrument mounts, it’s not without its challenges. Successfully implementing druk 3D z metalu for flight-critical hardware requires a deep understanding of potential pitfalls and robust strategies to mitigate them. Engineers and procurement managers should be aware of these hurdles when evaluating AM solutions and selecting manufacturing partners.
- Residual Stress and Distortion:
- Wyzwanie: The intense localized heating from the laser or electron beam, followed by rapid cooling, creates steep thermal gradients within the part during the build. This leads to the buildup of internal residual stresses. If these stresses exceed the material’s yield strength at elevated temperature, they can cause the part to warp or distort during the build, upon removal from the build plate, or even during post-processing (like machining). Residual stress AM jest głównym problemem.
- Mitigation:
- Process Simulation: Using simulation software to predict thermal history and stress accumulation helps optimize build orientation and support strategies before printing.
- Optimized Scan Strategies: Techniques like island scanning or checkerboard patterns can help manage heat distribution.
- Build Plate Heating: Maintaining an elevated temperature in the build chamber (especially common in EBM) reduces thermal gradients.
- Robust Support Structures: Well-designed supports anchor the part effectively and help conduct heat away.
- Post-Build Stress Relief: A critical heat treatment step performed immediately after printing, often before support removal, to relax internal stresses.
- Design Modifications: Avoiding large, abrupt changes in cross-section can help.
- Kontrola porowatości:
- Wyzwanie: Internal voids or pores within the printed material can act as crack initiation sites, significantly reducing fatigue life, ductility, and overall structural integrity. Porosity is one of the most critical powder bed fusion defects. Sources include:
- Gas Porosity: Trapped inert gas (from the build atmosphere) within the melt pool.
- Lack-of-Fusion Porosity: Insufficient energy input or improper beam/laser overlap leading to incomplete melting between layers or adjacent scan tracks.
- Powder Quality Issues: Hollow powder particles or contaminants.
- Mitigation:
- Optimized Process Parameters: Careful development and control of laser/beam power, scan speed, layer thickness, hatch spacing, and atmospheric conditions are crucial. Expertise from providers like Met3dp in parameter development is key.
- Powder Quality Control: Using high-quality, spherical powders with low internal gas content and consistent particle size distribution, like those produced by Met3dp’s advanced atomization systems. Strict powder handling protocols are essential.
- Prasowanie izostatyczne na gorąco (HIP): Very effective at closing internal pores (both gas and lack-of-fusion types) through high temperature and pressure, significantly improving density and mechanical properties. Often mandatory for critical parts.
- Badania nieniszczące (NDT): Using CT scanning to detect and quantify internal porosity is essential for quality assurance.
- Wyzwanie: Internal voids or pores within the printed material can act as crack initiation sites, significantly reducing fatigue life, ductility, and overall structural integrity. Porosity is one of the most critical powder bed fusion defects. Sources include:
- Powder Management, Handling, and Traceability:
- Wyzwanie: Metal powders, especially reactive ones like Ti-6Al-4V or fine aluminum alloys, require careful handling to prevent contamination (e.g., oxygen pickup, cross-contamination between alloys) and ensure safety (flammability/explosivity risks). Maintaining batch-to-batch consistency and full traceability from raw material to final part is critical for aerospace applications.
- Mitigation:
- Controlled Environments: Handling powders in inert glove boxes or dedicated controlled rooms.
- Strict Protocols: Documented procedures for powder loading/unloading, sieving, storage, and recycling.
- Charakterystyka proszku: Regular testing of powder properties (PSD, morphology, chemistry, flowability). Met3dp’s expertise starts with its advanced powder making system.
- Dedicated Equipment: Avoiding cross-contamination by using dedicated machinery or rigorous cleaning procedures between different materials.
- Batch Tracking: Implementing robust systems for tracking powder lots throughout the manufacturing process. Metal powder handling aerospace standards are stringent.
- Material Property Consistency and Repeatability:
- Wyzwanie: Ensuring that mechanical properties (strength, ductility, fatigue life) are consistent within a single part, from part-to-part within a build, and from build-to-build over time can be challenging due to the sensitivity of AM processes to numerous variables.
- Mitigation:
- Process Monitoring & Control: Utilizing in-situ monitoring tools (melt pool monitoring, thermal imaging) and tightly controlling all process parameters (laser power, gas flow, temperature, etc.).
- Machine Calibration: Regular and thorough calibration of AM systems.
- Standardized Procedures: Operating according to well-documented and validated procedures.
- Consistent Feedstock: Using powders with consistent quality and properties batch after batch.
- Witness Coupons: Including test specimens (witness coupons) within builds, which are then destructively tested to verify the mechanical properties achieved in that specific build cycle.
- Kwalifikacje i certyfikacja:
- Wyzwanie: Additive manufacturing is still relatively new compared to traditional methods like forging or machining. Establishing the necessary process maturity, repeatability, and documentation to meet stringent aerospace qualification and certification requirements (e.g., AS9100 additive manufacturing standards, specific NASA/ESA/FAA requirements) is a significant undertaking. Proving equivalence or superiority to legacy processes often requires extensive testing and analysis.
- Mitigation:
- Developing Process Specifications: Creating detailed specifications that define all aspects of the manufacturing process, from powder handling to final inspection.
- Statistical Process Control (SPC): Implementing SPC to monitor process stability and capability.
- Extensive Testing: Performing comprehensive material characterization (static, dynamic, fatigue testing) on parts built under the defined process.
- Robust Quality Management System (QMS): Operating under a certified QMS like AS9100.
- Collaboration: Working closely with certification agencies and customers throughout the qualification process. AM process qualification is often a joint effort.
Overcoming these challenges requires significant investment in technology, process control, materials science expertise, rigorous quality management, and skilled personnel. Partnering with an experienced and capable metal AM provider like Met3dp, which possesses deep expertise in materials, processes like SEBM, powder production, and quality assurance, is critical for successfully navigating these complexities and realizing the full benefits of additive manufacturing for demanding spacecraft applications.
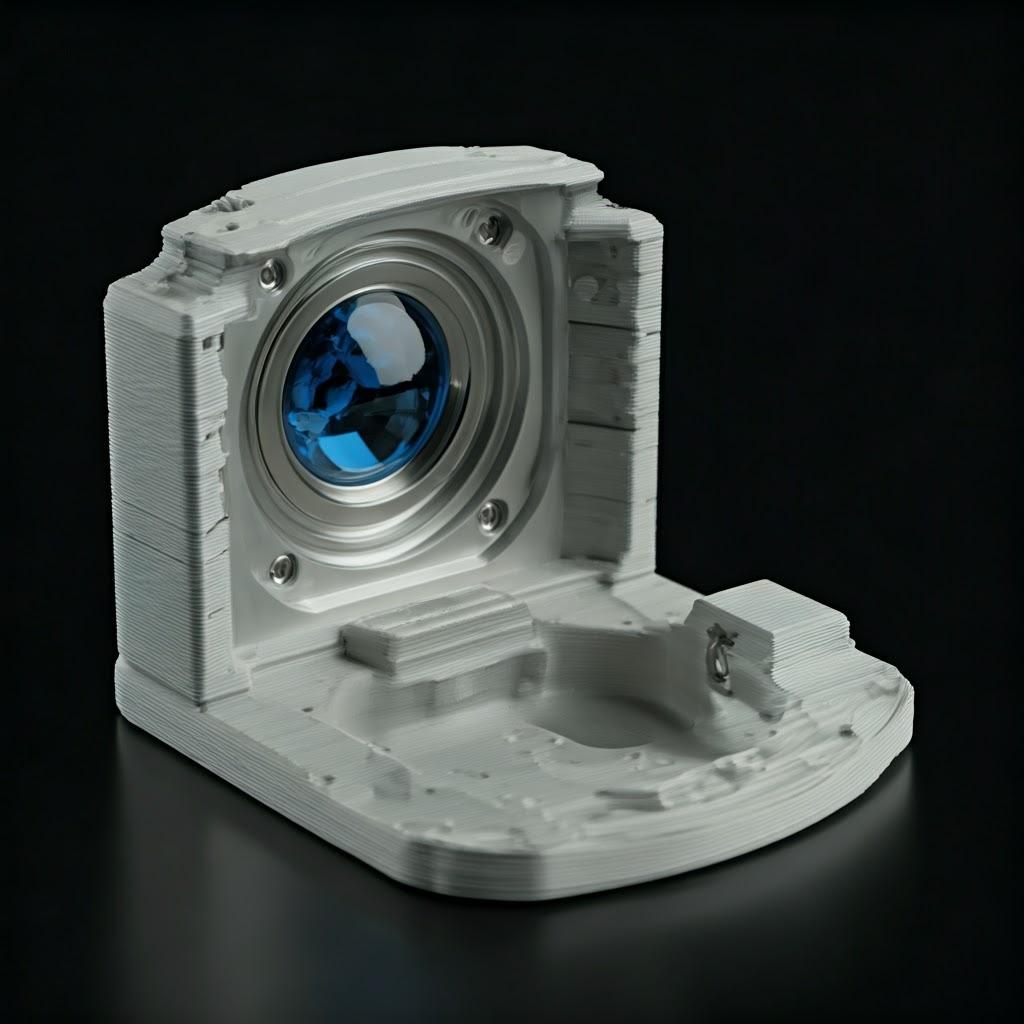
Selecting Your Metal Additive Manufacturing Partner for Space-Grade Components
Choosing the right manufacturing partner is arguably one of the most critical decisions when implementing metal additive manufacturing for demanding applications like spacecraft instrument mounts. The difference between success and failure often hinges on the partner’s expertise, capabilities, quality systems, and collaborative approach. This is not merely a transactional purchase; it’s selecting a partner invested in delivering flight-worthy hardware. For procurement managers and lead engineers evaluating metal AM service provider aerospace options, a structured evaluation process focusing on specific criteria is essential.
Here’s a checklist of key factors to consider when selecting your aerospace 3D printing supplier:
- Demonstrable Aerospace Experience & Track Record:
- Proven Success: Has the provider successfully manufactured components for similar aerospace or defense applications? Can they share relevant (non-proprietary) case studies or examples?
- Industry Understanding: Do they understand the unique requirements, standards, and challenges of the space industry (e.g., environmental conditions, reliability demands, cleanliness standards)?
- ITAR Compliance (if applicable): If dealing with defense-related projects subject to US International Traffic in Arms Regulations, ensure the provider is ITAR registered and compliant.
- Quality Management System & Certifications:
- AS9100 Certification: This is the standard QMS requirement for the aviation, space, and defense industries. Look for AS9100 certified AM providers. This demonstrates a commitment to rigorous process control, traceability, risk management, and continuous improvement aligned with aerospace expectations.
- Robust QMS: Even beyond AS9100, evaluate the maturity and implementation of their overall Quality Management System. How do they handle non-conformances, corrective actions, document control, and configuration management?
- Material Traceability: Can they demonstrate full traceability for metal powders, from the initial powder batch certification through processing, recycling (if used), and linking to the final part serial number?
- Material Expertise and Handling:
- Specific Alloy Experience: Do they have deep experience processing the specific alloys required (e.g., Scalmalloy printing service, Ti-6Al-4V printing service)? This includes validated parameter sets, understanding of microstructure development, and appropriate post-processing knowledge.
- Powder Quality & Management: What are their procedures for incoming powder inspection, storage, handling (especially for reactive metals), sieving/recycling, and preventing cross-contamination? Do they source high-quality powders or, like Met3dp, produce their own with advanced techniques?
- Broader Material Portfolio: While you may need specific alloys now, does the provider work with a range of relevant aerospace materials (e.g., Inconel, other titanium grades, stainless steels)? This indicates broader expertise. Met3dp, for instance, manufactures powders like TiNi, TiTa, TiAl, TiNbZr, CoCrMo, stainless steels, and superalloys.
- Technical Capabilities & Equipment:
- Process Expertise (L-PBF, EBM, etc.): Do they possess the right AM technology (e.g., Laser Powder Bed Fusion, Electron Beam Melting) suitable for your material and application? Do they have mastery over the process parameters?
- Machine Quality & Calibration: Are their AM machines well-maintained, regularly calibrated, and suitable for producing high-quality aerospace parts? What is the condition and age of their fleet? Met3dp prides itself on printers delivering industry-leading print volume, accuracy, and reliability.
- Objętość kompilacji: Can their machines accommodate the size of your instrument mount?
- Environmental Controls: Are build chambers properly controlled (inert atmosphere quality, temperature) to ensure material integrity?
- Engineering and DfAM Support:
- Collaborative Approach: Are they willing and able to work collaboratively with your design team? Can they provide expert DfAM feedback to optimize the design for printability, performance, and cost-effectiveness?
- Simulation Capabilities: Do they utilize process simulation tools to predict and mitigate potential issues like distortion?
- Problem-Solving Skills: How do they approach technical challenges during development or production?
- Post-Processing Capabilities:
- In-House vs. Outsourced: Do they offer critical post-processing steps (stress relief, HIP, machining, finishing, cleaning) in-house, or do they manage a network of qualified subcontractors? In-house capabilities often streamline workflow and improve quality control.
- Quality of Post-Processing: Ensure their post-processing methods meet aerospace standards, particularly for heat treatment (calibrated furnaces, proper atmospheres) and CNC machining (appropriate machines, experienced machinists).
- Inspection and Metrology:
- Comprehensive Capabilities: Do they have the necessary equipment (CMM, 3D scanners, surface profilometers) and expertise for thorough dimensional inspection according to your drawings and GD&T requirements?
- NDT Capabilities: Crucially, can they perform or manage required Non-Destructive Testing, especially CT scanning for internal defect detection in critical AM parts?
- Capacity, Lead Time, and Communication:
- Zdolność produkcyjna: Can they handle your required volume and meet your project timelines? What are their typical lead times?
- Responsiveness & Communication: Are they responsive to inquiries and transparent in their communication throughout the project lifecycle? Who will be your primary point of contact?
- Konkurencyjność kosztowa:
- Value Proposition: While cost is always a factor, evaluate it in the context of the provider’s expertise, quality, capabilities, and overall value proposition, not just the lowest price. A cheaper part that fails qualification or in service is infinitely more expensive.
Choosing AM partner is about building trust and ensuring capability. Look for evidence supporting claims in each of these areas. Site audits, reference checks, and pilot projects can be valuable steps in the selection process. Companies like Met3dp, with decades of collective expertise and Met3dp’s comprehensive solutions spanning advanced SEBM printers, high-performance metal powders produced in-house via gas atomization and PREP, and dedicated application development services, represent the type of vertically integrated and knowledgeable partner needed for success in demanding aerospace additive manufacturing.
Understanding Cost Drivers and Lead Times for 3D Printed Mounts
While metal additive manufacturing offers significant performance and design advantages, understanding the factors that influence cost and lead time is crucial for project planning, budgeting, and making informed comparisons with traditional manufacturing methods. Both metal 3D printing cost analysis and realistic AM lead time estimation require considering multiple elements beyond just the raw material.
Key Cost Drivers in Metal AM:
- Koszt materiałów:
- Powder Price: The cost per kilogram of aerospace-grade metal powder is a significant factor. Materials like Scalmalloy cost (due to scandium) and Ti-6Al-4V cost are substantially higher than conventional aluminum or steel alloys. Prices can vary based on supplier, powder quality (e.g., sphericity, purity, PSD), and quantity purchased.
- Powder Consumption & Recycling: While AM is often near-net shape, some powder is consumed by supports, and some cannot be fully recovered or reused indefinitely. Effective powder recycling strategies employed by the AM provider can help mitigate costs, but strict quality control on recycled powder is essential. Buy-to-fly ratios are generally much better than machining complex parts but not negligible.
- Machine Utilization (Print Time):
- Machine Hourly Rate: AM machines represent significant capital investment and have operational costs (power, inert gas, maintenance). Providers typically charge based on the time the machine is occupied printing the part.
- Build Height & Volume: Build time is primarily driven by the height of the part (number of layers) but also influenced by the volume/area to be scanned per layer. Taller parts take longer.
- Part Density & Complexity: Solid, dense parts take longer to scan than those incorporating significant lightweighting (e.g., lattices). Highly complex geometries may require slower scan speeds for certain features.
- Number of Lasers/Beams: Multi-laser/beam machines can process layers faster, potentially reducing print time for larger parts or multiple parts per build.
- Grubość warstwy: Thinner layers improve resolution and surface finish on slopes but increase the total number of layers and thus print time.
- Engineering & Design Effort:
- DfAM & Optimization: Time spent by engineers optimizing the design for additive manufacturing (topology optimization, lattice generation, support strategy) contributes to the overall cost, especially for novel or highly complex parts.
- Build Preparation & Simulation: Setting up the build file, determining optimal orientation, generating support structures, and potentially running process simulations adds to the upfront engineering cost.
- Post-Processing Complexity & Labor:
- Usuwanie wsparcia: Labor-intensive, especially for complex parts or tough materials. Can require skilled technicians and specialized tools (e.g., EDM).
- Heat Treatment (Stress Relief, HIP, Aging): Requires furnace time, energy, controlled atmospheres, and associated labor. HIP is a particularly specialized and costly process.
- CNC Machining: Costs depend on the amount of material to be removed, the number of features requiring machining, the required tolerances, and CNC machine time/labor rates.
- Wykończenie powierzchni: Costs vary significantly depending on the method (blasting is relatively cheap, extensive polishing is expensive) and the required final Ra value.
- Cleaning & Inspection: Labor and equipment time for rigorous cleaning protocols, CMM measurements, 3D scanning, NDT (especially CT scanning), and documentation add to the cost.
- Qualification & Testing:
- NDT Costs: CT scanning, FPI (Fluorescent Penetrant Inspection), or other required NDT methods add cost.
- Destructive Testing: If witness coupons or sample parts require destructive testing (tensile, fatigue, metallography) as part of the qualification process, these laboratory analysis costs must be included.
- Quantity & Batch Size:
- Korzyści skali: Setting up a build involves fixed overheads. Printing multiple parts in a single build (if geometry and build volume allow) spreads these setup costs, reducing the cost per part 3D printing. Larger production runs generally allow for greater efficiency and potentially lower per-part costs compared to one-off prototypes, although AM is cost-effective even for single units compared to tooling-intensive traditional methods. Wholesale 3D printing cost inquiries should specify expected volumes.
Typical Lead Times:
One of the most significant advantages of AM is often speed, particularly compared to processes requiring tooling (casting, forging) or extensive machining setups for complex parts.
- Proces AM: Printing itself can take anywhere from several hours for small parts to several days or even a week+ for very large, complex components or full build plates.
- Przetwarzanie końcowe: This often constitutes a significant portion of the total lead time. Heat treatments take time (including controlled cooling cycles). Support removal and machining can be time-consuming depending on complexity. HIP requires scheduling with specialized facilities if not available in-house.
- Overall:
- Prototypes/Simple Parts: Lead times of 1-3 weeks are often achievable.
- Complex/Production Parts: Including full post-processing and inspection, lead times of 4-8 weeks are common for qualified aerospace components. This is still often considerably faster than the months potentially required for traditional manufacturing routes involving tooling procurement or complex supply chains.
- Factors Influencing Lead Time: Part complexity, material choice, the specific post-processing steps required, machine availability (queue times at the provider), testing requirements, and documentation needs all impact the final delivery schedule.
Clear communication with the AM provider regarding all requirements (tolerances, finish, testing, standards) is essential for obtaining accurate additive manufacturing pricing and realistic lead time estimates.
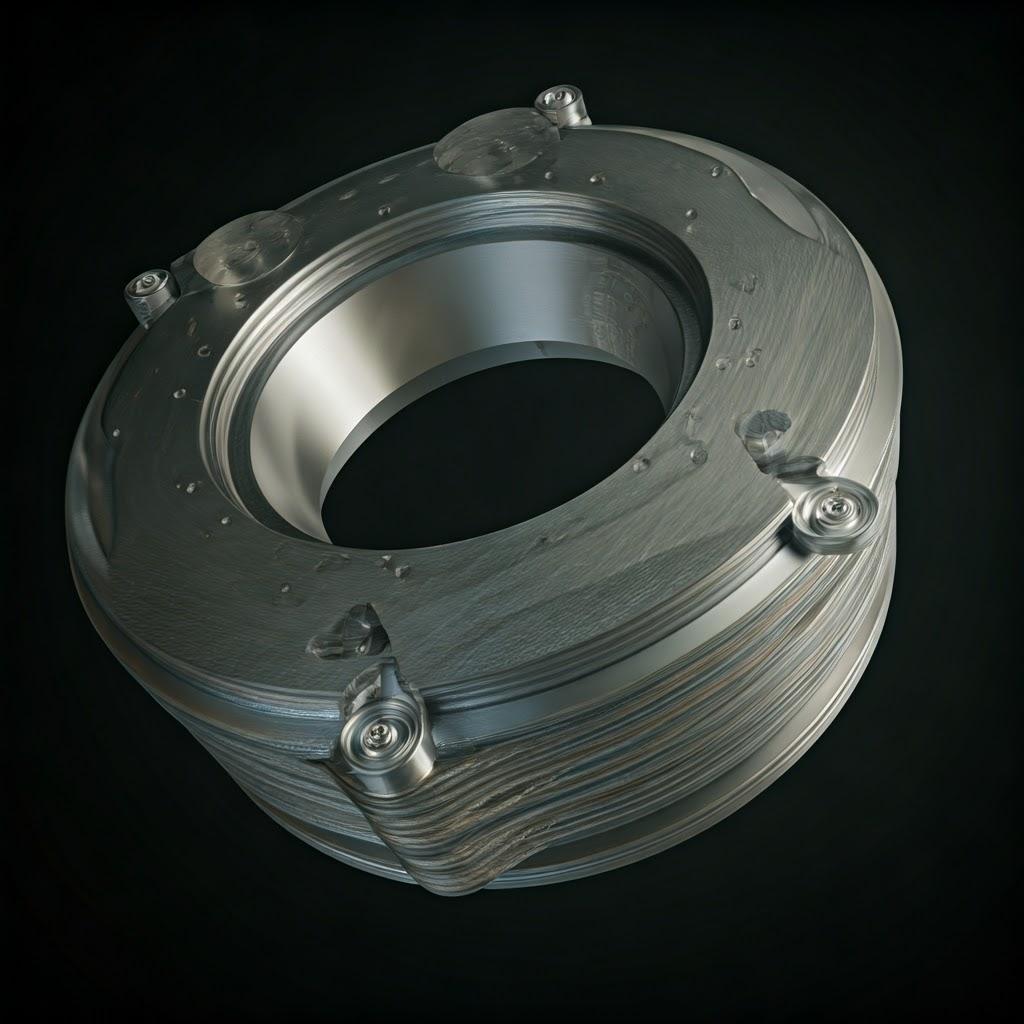
Często zadawane pytania (FAQ)
Here are answers to some common questions engineers and procurement managers have about using metal additive manufacturing for spacecraft instrument mounts:
- Q1: What kind of weight savings can realistically be achieved for instrument mounts using AM compared to traditional machining?
- A: Significant weight savings are often achievable, typically ranging from 20% to 50%, and sometimes even more. The exact savings depend heavily on the original design, the functional requirements, and the extent to which DfAM principles like topology optimization and lattice structures are employed. For components not previously optimized for weight, the potential savings are often substantial, directly impacting launch costs and potentially enabling increased payload capacity.
- Q2: How does the structural integrity and fatigue life of 3D printed Scalmalloy® or Ti-6Al-4V compare to wrought or cast counterparts?
- A: When produced using optimized process parameters and appropriate post-processing (especially stress relief and HIP), the mechanical properties of AM Scalmalloy® and Ti-6Al-4V can meet or even exceed those of traditional cast materials and often approach or match wrought properties, particularly in terms of specific strength (strength-to-weight ratio). HIP is crucial for reducing internal porosity, leading to fatigue properties that are often comparable to wrought material, making them suitable for demanding, cyclically loaded aerospace applications. However, properties can be anisotropic (direction-dependent), requiring careful consideration during design and qualification testing.
- Q3: What are the critical quality control and inspection steps required for flight-critical 3D printed parts?
- A: Quality control is paramount. Key steps typically include:
- Powder Batch Certification: Verifying chemistry, PSD, and morphology of the incoming metal powder.
- Process Monitoring: In-situ monitoring of the build process where available.
- Post-Build Inspection: Visual inspection, dimensional checks (CMM, 3D scanning) against GD&T requirements.
- Badania nieniszczące (NDT): Critically, CT scanning is often required to ensure internal soundness and detect defects like porosity or lack of fusion. Surface crack detection methods like FPI may also be used after machining.
- Material Testing: Destructive testing (tensile, fatigue, hardness, metallography) performed on witness coupons built alongside the parts to verify material properties achieved in that specific build cycle.
- Documentation: Rigorous documentation tracking materials, processes, inspections, and test results is essential for flight certification.
- A: Quality control is paramount. Key steps typically include:
- Q4: Can complex internal channels for thermal management or fluid flow be integrated directly into the 3D printed mount?
- A: Yes, absolutely. This is one of the major advantages of additive manufacturing. AM allows the creation of intricate internal passages and conformal cooling/heating channels that follow the contours of the instrument or heat source precisely. These geometries are often impossible or prohibitively expensive to produce using traditional methods like drilling or machining. This enables highly efficient thermal management solutions integrated directly into the structural mount, saving weight and improving performance. Careful design (DfAM) is needed to ensure powder removal from these channels.
- Q5: What information does a metal 3D printing service provider like Met3dp need to provide an accurate quote for an instrument mount?
- A: To provide an accurate quote and manufacturability assessment, the provider typically needs:
- Model 3D CAD: Preferably in STEP (.stp/.step) format. Native CAD files or mesh files (STL) can sometimes be used but STEP is generally preferred for interrogation.
- Material Specification: Clearly defined alloy (e.g., Ti-6Al-4V Grade 5, Scalmalloy®) and any specific material standards (e.g., ASTM, AMS).
- 2D Drawings (if applicable): Essential if specific Geometric Dimensioning and Tolerancing (GD&T), critical tolerances, surface finish requirements, or inspection criteria are defined that aren’t fully captured in the 3D model.
- Required Tolerances: Indication of general tolerances and highlighting of any critical dimensions requiring tighter control (which may imply post-machining).
- Wymagania dotyczące wykończenia powierzchni: Specify required Ra values or surface treatments for specific features or the entire part.
- Potrzeby przetwarzania końcowego: Specify required heat treatments (stress relief, HIP, aging), machining, finishing, and cleaning/passivation requirements.
- Ilość: Number of parts required (prototype vs. production volumes).
- Required Certifications/Standards: Any specific industry standards (e.g., AS9100 compliance) or customer-specific quality requirements.
- Testing & Inspection Requirements: Detail any mandatory NDT or destructive testing.
- A: To provide an accurate quote and manufacturability assessment, the provider typically needs:
Providing comprehensive information upfront allows the B2B additive manufacturing provider to give the most accurate cost estimate, lead time projection, and feedback on design feasibility.
Conclusion: Launching the Future with 3D Printed Spacecraft Instrument Mounts
The journey to space is inherently challenging, demanding components that offer exceptional performance, unwavering reliability, and minimal mass. As we’ve explored, spacecraft instrument mounts are critical interfaces that directly impact mission success by ensuring the stability and function of sensitive payloads. Metal additive manufacturing has emerged as a disruptive force, fundamentally changing how these vital components are designed and produced.
By embracing technologies like Laser Powder Bed Fusion and Electron Beam Melting, and leveraging advanced materials like Scalmalloy® oraz Ti-6Al-4V, the aerospace industry can realize transformative benefits:
- Unprecedented Lightweighting: Topology optimization and lattice structures enabled by AM drastically reduce component mass, lowering launch costs and freeing up capacity for more functional payloads.
- Geometric Freedom: Engineers can design highly complex, integrated structures optimized for performance, incorporating features like conformal cooling or intricate load paths impossible with traditional methods.
- Konsolidacja części: Reducing assembly complexity, weight, and potential failure points by printing multiple components as one monolithic part.
- Zwiększona wydajność: Tailored stiffness, improved vibration damping, and optimized thermal management contribute to better instrument performance and stability.
- Accelerated Timelines: Rapid prototyping and faster production cycles compared to tooling-dependent traditional manufacturing shorten development and deployment schedules.
The future of aerospace manufacturing is increasingly intertwined with additive processes. For spacecraft instrument mounts, metal AM is not just an alternative; it’s often the superior solution for meeting the conflicting demands of extreme performance and minimal weight inherent in space applications.
Successfully navigating the complexities of AM – from Design for Additive Manufacturing (DfAM) principles and material selection to intricate post-processing pathways and rigorous quality assurance – requires expertise and capable partners. Companies like Met3dp, with a foundation built on decades of collective expertise in metal additive manufacturing, stand ready to help organizations harness the power of this technology. Our comprehensive capabilities, spanning advanced SEBM printers with industry-leading accuracy, high-quality metal powders manufactured in-house using cutting-edge Gas Atomization and PREP technologies, and dedicated application development support, provide a complete solution package.
We understand the demanding requirements of the aerospace sector and partner with organizations to implement 3D printing effectively, accelerating digital manufacturing transformations. Explore how Met3dp’s advanced metody drukowania and superior materials can elevate your next space mission.
Contact Met3dp today to discuss your specific spacecraft component needs and discover how our cutting-edge systems and powders can power your organization’s additive manufacturing goals, launching your projects to new heights.
Udostępnij
MET3DP Technology Co., LTD jest wiodącym dostawcą rozwiązań w zakresie produkcji addytywnej z siedzibą w Qingdao w Chinach. Nasza firma specjalizuje się w sprzęcie do druku 3D i wysokowydajnych proszkach metali do zastosowań przemysłowych.
Zapytaj o najlepszą cenę i spersonalizowane rozwiązanie dla Twojej firmy!
Powiązane artykuły
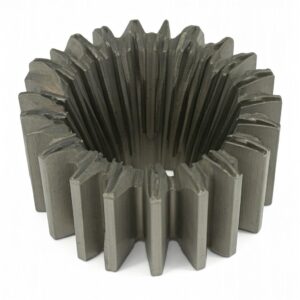
Wysokowydajne segmenty łopatek dysz: Rewolucja w wydajności turbin dzięki drukowi 3D w metalu
Czytaj więcej "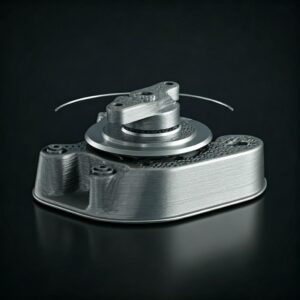
Drukowane w 3D mocowania dla samochodowych czujników radarowych: Precyzja i wydajność
Czytaj więcej "Informacje o Met3DP
Ostatnia aktualizacja
Nasz produkt
KONTAKT
Masz pytania? Wyślij nam wiadomość teraz! Po otrzymaniu wiadomości obsłużymy Twoją prośbę całym zespołem.
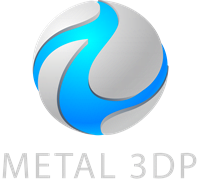
Proszki metali do druku 3D i produkcji addytywnej